Intraneuronal Computation: Charting the Signaling Pathways of the Neuron
Jorge Navarro, Raquel del Moral, and Pedro C. Marijuán
4.1 Introduction: The Centrality of Intracellular Signaling
A bewildering variety of signaling components and pathways co‐occur in neurons—more than in any other living tissue. During the 1970s and 1980s, perhaps the “golden age” of molecular neurobiology, the sheer variety of receptors, channels, transducers, protein kinases, and many other components discovered in neurons was seen as an “impenetrable jungle” (Crick, 1979). Neural tissue appeared special in many ways (Allman, 1999; John & Miklos, 1988): It consumes up to ten times as much metabolic energy as average, keeps the largest number of genes in an active state of expression, synthesizes the highest total amount of protein, and contains the highest number of specialized cell types—more than 100 in humans, over half our total, despite taking up merely 2% our body mass. What was so special about the evolutionary function of the neuron? Why were the molecular mechanisms involved so complex and so expensive? The pioneering discussions in that “golden age” about the function of the neuron and the origins of nervous systems may count among the most elegant debates in the modern history of neurobiology (Bullock & Horridge, 1965; Horridge, 1968; Mackie, 1990; Pavans de Ceccatty, 1974).
While it can be asked of any tissue how its specialized function is necessary to the multicellular organism, this question is particularly cogent in the extreme case of neuronal complexity. Roughly speaking, the tissular function of neurons deals with receiving signals via neurotransmitters and other sources, maintaining a transmembrane electrical potential, transporting the electrical disturbances of the membrane potential along dendrites and axonal prolongations, and maintaining a vast population of actualized synaptic contacts. In engineering terms, this implies a complex molecular management of both an extended electrical‐computational network and a distributed memory system. However, the molecular mechanisms and subsystems implementing these functions have been, and continue to be, highly elusive. When the concept of a cellular signaling system was established in early 1990s (Cohen, 1992; Egan & Weinberg, 1993), it became clear that the enormous variety of membrane and cytoplasmic neuronal mechanisms discovered during the two previous decades were caught up in that category. Moreover, signaling is the appropriate label to characterize the special function and complexity of neuronal tissues, as they express more (and more diverse) signaling elements than any other eukaryotic tissue or cellular specialization.
The complexity of signaling pathways expressed in neurons did not arise from scratch. A good portion of neurons’ signaling system was directly inherited from prokaryotes, but new additions were incorporated through bricolage, cobbled together through complex controlling apparatuses foreign to prokaryotes. Functionally speaking, however, the relative simplicity attributed to prokaryotic cells is only apparent, at least as far as their signaling capabilities are concerned. As will be discussed later, prokaryotic cells have only three main classes of “component‐system” arrangements for signaling purposes, but they are instantiated in about one or two hundred different pathways for each cell, acting as mostly independent channels for the introduction and processing of external information. In contrast, the cells of complex eukaryotes, such as vertebrates and mammals, are endowed with several dozen major classes of component‐system arrangements (main signaling pathways), but these comprise thousands of specific molecular implementations in different tissues, particularly within the nervous system. Amidst all that complexity, however, there is a deep evolutionary coherence in the way signaling pathways operate in eukaryote neurons. Making evolutionary sense of this diversity will be the goal of this chapter.
Genomic studies of transition from prokaryotes to eukaryotes and the origins of synaptic contacts are providing important new insights into the origins of nervous systems themselves (Miller, 2009) and into the evolution of advanced signaling control, with a good portion traceable to humble bacterial ancestors (Aravind, Anantharaman, & Iyer, 2003; Aravind, Iyer, & Koonin, 2006). In what follows, we will not depart from the basic idea that there is an informational continuum of signaling tools and strategies, and that any attempt to explain neuronal complexity must connect with the evolutionary trajectory of signaling systems and with the origins of nervous systems as one of the obligate, earliest inventions of eukaryotic multicellularity. A synthetic path must be established among a forest of intricate discoveries in systems biology, signaling science, synthetic biology, genomics, proteomics, molecular biology, evolutionary neurobiology, neurophysiology, and so on.
Two distributed memory systems were invented by metazoa: an immune system recognizing “molecular configurations” and a nervous system recognizing “sensorimotor configurations.” Both adapt the organism to an open‐ended and fast‐changing environment, and they maintain a continuous dialog between them (Arnsten, 2009; Steinman, 2004). It is intriguing that a good portion of their informational organization and molecular signaling cascades is still unknown. This brief chapter is a small step in bringing them out of the shadows: It is meant to serve as a synopsis of the basic eukaryotic pathways deployed in neurons and as an (admittedly speculative!) rationale for their evolution.
4.2 How Signaling Resources Evolved in the Transition from Prokaryotic to Eukaryotic
An information revolution took place in cellular systems around 1200 million years ago. It was preceded and made possible by an energy revolution derived from the symbiotic capture of mitochondria, as Lynn Margulis so forcefully argued in her theory of endosymbiosis (Margulis, 1970). The data are staggering: An average protozoan has nearly 5000 times more metabolic power than a single bacterium, supporting a genome several thousand times larger, with over two orders of magnitude more energy devoted to the expression and translation of each gene (Lane & Martin, 2010). Whereas prokaryotes had already made a start towards eukaryotic‐style cellular complexity, they could not exhibit more than one complex trait at a time, given the implicit energy costs. Novel protein foldings, protein interactions, and regulatory cascades were required to integrate the segregated traits already explored in bacteria—innovations including a separate nucleus, dynamic cytoskeleton, endocytosis, linear chromosomes, introns and exons, massive intracellular and intercellular signaling, etc. The increase in protein repertoire by the “last eukaryotic common ancestor” (LECA) was dramatic: It represented some 3,000 novel gene families—the most intense phase of gene invention since the origin of life: “If evolution works like a tinkerer, evolution with mitochondria works like a corps of engineers” (Lane & Martin, 2010, p. 933).
A heavy investment in signaling resources was necessary in order to produce a new kind of life cycle amenable to controlled dissociation or modularization amidst newly complex internal and external happenstances. Most cellular functions had to change from a temporal context to a spatial one, tightly controlled by signals: While some functions were delayed or directly suppressed, others became augmented and specialized (Nedelcu & Michod, 2004). The decoupling of cell division from genuine cell reproduction, organizing successive levels of differentiation potency during development, was one of the central achievements. The ability to govern cellular reproduction permissively or suppressively depending on signaling contexts made possible the advent of true multicellularity (Davidson, 2006; 2010).
Four main resources were used in the expansion of eukaryotic signaling systems, four “roots” that supported the fast elaboration of new eukaryote capabilities. Two were directly taken from existing prokaryotic stock:
- Prokaryotic signaling pathways devoted to detection of solutes, comprising receptors, protein kinases, phosphatases, and regulated transcription factors.
- Prokaryotic apparatuses for solvent sensing (which maintain osmotic homeostasis by counteracting the Donnan effect) comprising stretch‐gated ion channels, voltage‐gated ion channels, ligand‐gated channels, water transporters, and pumps.
Another two signaling avenues were related to new cellular subsystems supporting the enlarged eukaryotic complexity. Co‐opted for signaling purposes, these control apparatuses modularized the deployment of cellular functions and became facultative components of the emergent complex network of signaling pathways:
- The cell‐cycle control system, comprising hierarchies of protein kinases, checkpoints, cyclins, and protein degradation systems.
- The cytoskeleton and endocytic matrix, providing mechanical support, adhesion, and force‐field detection on the one side; compartments, inner transportation, and vesicle formation on the other.
Lynchpins of prokaryotic metabolism provided key substances previously involved in detecting the energetic state of the cell (cAMP, cGMP) and in the synthesis and integrity of membrane systems (IP3, DAG, arachidonic acid, ceramide acid), as well as the key ionic effector, Ca++. They were reused inside the eukaryotic signaling pathways as second messengers or “symbolic molecules” to amplify the information flow, conveying integrative messages by diffusing through localized regions of a far bigger cell, in connection to diverse membrane systems, compartments, and inner transportation mechanisms.
Before entering into analysis of these different signaling resources, either segregated or nonexistent in prokaryotic physiology, we must address the fundamental question: What genetic strategy made possible the assembly of eukaryotic signaling systems?
4.2.1 Recombination Is a Central Theme of Signaling System Evolution
The accelerated evolution of the new signaling systems was fundamentally based on protein‐domain recombination. The signaling innovations of eukaryotic cells, crucial to nervous systems, were not due to any of these previous “roots” in isolation, but rather to their integration into larger systems of cross‐linked pathways. Osmotic tools (i.e., ion channels) were liberally interwoven with protein receptors for solute detection, with hierarchical chains of protein kinases and with endosomal pathways for protein recycling culminating in ubiquitylation and degradation. The postsynaptic processing of the neurotransmitter glutamate is one of the best examples of how these heterogeneous signaling resources were recombined (as we will analyze later). The multidomain structure of most involved enzymes, proteins, channels, and receptors, in addition to the flexibility of their binding properties, made possible the evolution of interconnected pathways (Pawson & Nash, 2003). In particular, the arrangement of signaling components into integrated scaffolds, themselves subject to domain recombination, was a high‐level way to exploit both specificity and convergence in pathways.
Scaffold proteins avoid the entropic cost of diverse signaling molecules finding specific partners in a solution; they also afford easy regulation by external signals which modify the association of proteins along the scaffold, and so offer a simple, flexible strategy for regulating the selectivity of individual pathways, shaping signaling regimes and achieving new responses from preexisting components (Good, Zalatan, & Lim, 2011). Being themselves modular, composed of multiple interaction domains and tandem repeat proteins assembled through recombination, scaffolds provided an elegant evolutionary solution to the prokaryotic conflict between signaling specificity and the efficient coordination of information flow through intracellular networks. From an evolutionary perspective, scaffolds were a crucial functional element supporting signal‐cascade recombination and the integration of eukaryotic signaling systems.
However, basic aspects of early eukaryotic evolution remain poorly understood. Phylogenomic reconstructions show that the characteristic complexity of eukaryotes, both in structure and signaling pathways, arose without any apparent intermediate grades of complexity between the widely separated levels of organization (Koonin, 2010). Multicellularity in general, and nervous systems specifically, evolved quickly by redeploying and recombining the communication tools of unicellular eukaryotes such as yeast. Thus, most components regulating structural plasticity in the synaptic pathways of mammals have analogous roles in unicellular responses to environmental cues (ions, nutrients, repellents) and in pheromonal communication among unicellular eukaryotes (Emes et al., 2008). In point of fact, one of the major evolutionary challenges would be explaining the signaling system of the LECA. How did this system evolve, and how much of modern signaling systems are conserved from this common ancestor?
As new experimental studies and bioinformatic analyses of protein domain architecture have suggested, a number of bacterial and eukaryotic signaling proteins share similar mechanisms (Aravind et al., 2003, 2006; Koonin, 2010). In fact, the evidence suggests that laterally transferred domains of prokaryotic provenance (bacterial and archaeal) have contributed to the evolution of important sensory pathways related to stretch, light, nitric oxide, and redox signaling, as well as to central developmental pathways such as Notch, cytokine, and cytokinin signaling. The phagotrophic lifestyle of the LECA and of primitive eukaryotes could have served as a conduit for such lateral transfers, as well as for the endosymbiotic origins of mitochondria and chloroplasts themselves (Aravind et al., 2003, 2006). In terms of mechanisms (protein domains and architectures) the parallels between prokaryotic and eukaryotic signaling systems are startling, but it is in the flexible arrangement of functional modules and the intercombination of previously segregated systems where the major expansions of eukaryotic signaling complexity have occurred.
Of the four evolutionary forces acting on genes (mutation, recombination, selection, and drift), domain recombination seems to have been the prevailing creative force in eukaryotic signaling (Alm, Huang, & Arkin, 2006). Additional factors, such as intron–exon gene organization, differential splicing, and whole genome duplication (at least two instances) provided eukaryotes with far greater resources than prokaryotes to explore and leverage domain recombination. Taking into account that more than 45% of the eukaryotic genome is an accretion of residual transposons and retrotransposons, both ancient and recent, eukaryotes have explored quite a number of potential domain‐recombination events as well as multiple opportunities to fine‐tune their genomic directionality and control (Lander et al., 2001). The remarkable expansion of behavioral and cerebral complexity achieved by vertebrates is inseparable from the increasing sophistication achieved by their signaling systems through these evolutionary processes, augmented by the conservation and expansion of non‐coding cis DNA sequences devoted to fine‐tuning of gene control (Carroll, 2005; Davidson, 2010; Lynch et al., 2011). In the case of mammalian brains, recombination strategies seem to have been incorporated even deeper—in ontogenetic development. Recombination via retrotransposons and mobile elements that are active during cerebral development has become a new way to generate additional molecular complexity and mosaicism, particularly in the signaling capabilities of cortical and hippocampus´ neurons (Baillie et al., 2011; Kang et al., 2011; Lander et al., 2001).
We now examine in more detail the main resources and avenues—the “four evolutionary roots”—that eukaryotic signaling systems have put together, either through direct prokaryotic heredity or through functional co‐option and modularization.
4.3 Four Evolutionary Roots of Eukaryotic Signaling Systems
4.3.1 The Prokaryotic “Detection of Solutes”
The detection of external substances to be processed as signals (rather than as metabolic substrates) occurs in almost all prokaryotic cells. Indeed, it may well be considered the first evolutionary resource in the assemblage of eukaryotic signaling systems. In prokaryotes, a variety of molecular systems are involved in the detection of solutes, ranging from simple transcription‐sensory regulators (a single protein comprising two domains), such as the well‐known embR, alkA or furB, to systems of several components and to interconnected pathways that regulate key stages of the cell cycle, such as latency, pathogenesis, replication, and dispersion. We have proposed a basic taxonomy of bacterial signaling systems centering on “the 1‐2‐3 scheme” (Marijuán, Navarro, & del Moral, 2010) (see Figure 4.1.):
- The first level of signaling complexity corresponds to simple regulators, the “one‐component systems” (1CS). Actually, most cellular proteins involved in cellular adaptation to changing environments, in a general sense, could be included as participants in this primary category (Galperin, 2005). Around one hundred different 1CS elements may be present in a moderately complex prokaryotic cell.
- Increasing the scale of complexity, the “two‐component systems” (2CS) appear, which include a histidine kinase protein receptor and an independent response regulator. Conventionally, they are considered the central paradigm for prokaryotic signaling systems, and a number of intercellular communication processes between different prokaryotic and eukaryotic species are carried out by these specialized systems. A few dozen 2CS pathways may coexist in a prokaryote.
- To maintain conceptual coherence, an additional category, the “three‐component system” (3CS) should apply to two‐component systems that employ an additional non‐kinase receptor to activate a protein kinase. Very few prokaryotic pathways show a 3CS arrangement, but they are very important ones (e.g. chemotaxis) and are usually subject to further regulations, such as the variable methylation of receptors.

Figure 4.1 The Three Characteristic Signaling Pathways Developed by Prokaryotes.
The external stimulus is perceived either by an internal receptor–transducer (left part), or by a transmembrane histidine kinase that connects with a response regulator (middle), or by an independent receptor associated to the histidine kinase (right part).
Adapted from Marijuán 2010. Reproduced with permission of Elsevier.
The signaling costs and benefits of 1CS, 2CS, and 3CS systems determine their functional deployment. The relative disadvantage of 1CSs stems from the fact that they detect their stimuli almost exclusively in the cytosol (including environmental cues such as light, gases, and other small molecules); afterwards they act on DNA‐binding in more than 80% of cases (Grigoroudis, Panagiotidis, Lioliou, Vlassi, & Kyriakidis, 2007; Ulrich, Koonin, & Zhulin, 2005). The evolutionary strategy to overcome the limitations of these single signaling elements has consisted in dividing the individual protein in two, putting one half on the membrane while the other remains a soluble cytosolic regulator, and keeping both linked via a phosphotransfer relay (Ulrich et al., 2005). Two‐component systems (2CSs) thus arise as a good evolutionary solution enlarging signaling performance (sensitivity, amplification, adaptability) beyond the capabilities of single cytosolic receptor–transducers (1CS).
However, it must be recognized that the multitude of single proteins acting as 1CSs comprise the best‐represented signaling strategy within many bacteria, both pathogenic and free‐living. Moreover, 1CSs are the most primitive components endowed with signaling functions, their signaling dynamics are easily circulated among the multiple metabolic and transcriptional circuits, and they may straightforwardly interact with more complex signaling pathways. Thus, 1CS become a fundamental factor in the organization of “bacterial intelligence.” In point of fact, the relative predominance of 1CS cytosolic detection versus 2CS extracellular detection has been used to discriminate “introverted” from “extroverted” prokaryotes: those that are focused on controlling the inner metabolic complexity versus those that predominantly monitor the rapidly changing external environment. That ratio is also a way to gauge the extra metabolic complexities incorporated in the life cycle of the prokaryote (Galperin, 2005).
In the transition to eukaryotic signaling, most simple 1CSs were relegated to strictly metabolic functions; however, cytosolic and nuclear detection by single proteins remains the primary intracellular mechanism for steroid and hormone signaling, as well as other cases where specificity and security in molecular recognition become essential. While few 2CSs remain active in eukaryotes (mostly plants and yeasts), they have been massively replaced in animals by serine/threonine and autophosphorylated tyrosine receptors, which are very rare in prokaryotes. The reason is that phosphorylation by histidine protein kinases involves recognition of a 3‐dimensional folded surface, and is thus less amenable to recombination than serine/threonine kinases, which additionally recognize unstructured linear motifs (Kiel, Yus, & Serrano, 2010); similar evolutionary flexibility favors tyrosine kinases over 2CSs. Unconventional 2CSs do exist in bacteria: ECF proteins (related to “sigma factors”) and some eukaryotic‐like serine/threonine protein kinases and phosphatases that are used mainly to control cell shape and to manipulate the signaling pathways of eukaryotic host organisms. Interestingly, there seem to be a significant number of 3CSs among the eukaryotic‐like serine/threonine protein kinases present in many pathogenic bacteria.
As we will see in the pathways of Table 4.1. (§4.4.2), the machinery of solute detection, as adopted by eukaryotes, builds directly off 2CS and 3CS. In particular, ligand binding by transmembrane receptors and ion channels is of outmost importance for information processing in nervous systems.
Table 4.1 The Major Eukaryotic Signaling Pathways (21 of them), Highlighting the Main Mechanisms and Functionalities.
Signaling system pathways | Components (to DNA‐binding) | Main functions | References |
Early Development and Morphogenesis | |||
Wnt pathway (Glycoproteins) | Frizzled and LDL co‐receptors → β‐catenin→ TCF/LEF Non‐canonical: Wnt/Ca2+, Wnt/PCP | Cell proliferation, migration, polarity, neural differentiation, axonal growth. Ciliogenesis, craniofacialdevelopment and regeneration. | Dale, Sisson, & Topczewski, 2009 Eisenmann, 2005 Reya & Clevers, 2005 |
Hedgehog pathway (Hh proteins) | Ptc/Ptch1 → 7TM co‐receptor Smo→Ci/Gli | Cell growth, tissue homeostasis, neural tube differentiation, loss of neural stem cells, embryonic formation, tumor initiation and growth. | Bermann et al., 2002Hausmann, von Mering, & Basler, 2009 Lum & Beachy, 2004 |
Notch pathway (Notch/Delta proteins) | DSL TM proteins→ NICD → CBF1/CSL/Rbp‐j → MAML1 | Cell‐fate specification, differentiation, self‐renewal, proliferation, and apoptosis, stem cells maintenance, vertebrate segmentation. | Aulehla & Herrman, 2004 Ersvaer, Hatfield, Reikvam, & Bruserud., 2011 Schwanbeck, Martini, Bernoth, & Just, 2010 |
TGFβ pathway (TGFβ superfamily proteins) | Ser/Treo kinase (type I y II) →SMAD cascade Non‐canonical: Ras/MAPKs (ERKs & SAPKs) | Cell division, differentiation, migration and adhesion; neuronal development and remodeling, synapse formation and growth, programmed cell death, suppressor of carcinogenesis. | Derynck & Zhang, 2003 Massagué & Gomis, 2006 Mulder, 2000 Roberts & Mishra, 2005 |
Trk kinase pathway (neurotrophins) | p75NTR→Small G proteins →Ras/MAPK Non‐canonical: PLC, PI3K | Cell survival, proliferation, axon and dendrite growth and patterning, cytoskeleton assembly & remodeling, synaptic strength and plasticity. | Huang & Reichardt, 2003 Reichardt, 2006 Segal, 2003 |
Mid‐development and Organogenesis | |||
Integrin pathway (Glycoprotein) | ECM proteins → FAK →Ras/Raf→MEK → ERK Non‐canonical: actin, JNK, AKT/PKB, RLC | Differentiation, proliferation, cell shape and migration, cytoeskeletal organization, maintenance and cell survival. | Martin et al., 2002 Moser, Legate, Zent, & Fässler, 2009 Stevens & George, 2005 Yee, Weaver, & Hammer, 2008 |
Cadherin pathway (Glycoprotein) | PDZ‐domain proteins → protein phosphatase → protein kinase → actin Non‐canonical: Rho family GTPases, Wnt, RTK | Embryonic development, tissue morphogenesis and homeostasis. Synaptogenesis, synaptic plasticity and synapse morfogenesis. | Arikkath & Reichardt, 2008 Hulpiau & van Roy, 2009 Wheelock & Johnson, 2003Yagi & Takeichi, 2011 |
Nuclear hormone receptor pathway | NHR (monomer/homodimer/RXRheterodimer) → HREs | Cell growth and cycle progression, apoptosis, hypothalamic–pituitary–adrenal axis, neuro‐endocrine stress response, autonomic nervous system, development, metabolic homeostasis. | Aranda & Pascual, 2001 Beildeck, Gelmann, & Byers, 2010 |
Reelin pathway (Glycoprotein) | ApoER2/VLDLR → DAB1 → SFK → NMDAR Non‐canonical: Cdk5, BLBP/Notch1 | Neuronal migration and positioning in the developing brain, modulation of synaptic plasticity, induction and maintenance of LTP, stimulation of dendrites and dendrites spine, migration of neuroblast and neurogenesis. | Akopians et al., 2008 Chameau et al., 2009 Durakoglugil, Chen, White, Kavalali, & Herz, 2009 Niu, Yabut, & D’Arcangelo, 2008 |
Tissue Physiology | |||
Guanylatecyclase pathway (hormones, toxins, free radicals, calcium..) | GCs+NO→GMPc→ PK/PDE cascades GCp→GMPc→ PK/PDE cascades | Neuronal signal transduction, vascular smooth muscle relaxation, inhibition of platelet aggregation, electrolytic homeostasis. | Lucas et al., 2000 Hofmann, Feil, Kleppisch, & Schlossmann, 2006 |
G‐protein coupled receptor (large G proteins) pathways | Adenyl cyclase→ cAMP → EPACs/PKA/CNG channels GPCRs+ Src‐related Kinase + PI3K+ Shc → Ras →MAPK | Cellular responses to hormones and neurotransmitters, immune responses, cardiac and smooth muscle contraction and blood pressure regulation, proliferation, tissue remodeling and repair, inflammation, angiogenesis, normal cell growth and cancer. | Dorsam & Gutkind, 2007 Gavi, Shumay, Wang, & Malbon, 2006 Rosenbaum et al., 2009 |
Electrical Transmission | |||
Gap junctions | Hemichannels or connexones permanently opened allowing unspecific ionic flow. | Direct electrical transmission between neurons (and also glial cells). | Kandel, Schwartz, & Jessell, 2000 Kelsell, Dunlop, & Hodgins, 2001 Willecke et al., 2002 |
Stretch‐activated channels | Mechanotransducer channels sensing membrane stress and allowing non‐specific ionic flow. | Vibration sensing, pressure, stretch, touch, heat sensation, hearing, osmotic and blood pressure, and propioceptive sensation. | Kung, 2005 Purves et al., 2008Yin and Kuebler, 2010 |
Voltage‐activated channels | Voltage sensor channels allowing specific ionic flow (sodium, potassium, calcium, chloride, proton). | Generation and transmission of electrical signals in central and peripheral neurons, glia, skeletal muscle, heart, kidney, vascular tone, hormonal control, etc. | Alberts et al., 2002 Catterall, 2000 Kandel et al., 2000 |
Ligand‐gated channels | Transmembrane ion channels opened or closed in response to the binding of a ligand or neurotransmitter, and allowing selective ionic flow (sodium, potassium, calcium, chloride, proton). | The main neurotransmitters (glutamate, acetylcholine, GABA, glycine, serotonin, etc.) represent the basic controls of brain function and the most general means of communication between neurons. | Barry & Lynch, 2005 Kandel et al., 2000 Swijsen, Hoogland, & Rigo, 2009 |
Stress and Criticality | |||
Toll‐like receptors pathway | TLR(1‐2, 4‐13) MyD88dependent+ IRAK kinases → TRAF6 → TAK1 →IKKs →NFκB TLR (3‐4) TRIF dependent+ TBK/RIP1→ IRF3/TAK1‐NFκB | Apoptosis, cell mediated immunity, bacterial death, autoimmune diseases (inflammatory process). | Kumar, Kawai, & Akira, 2009 O’Neill, Fitzgerald, & Bowie, 2003 O’Neill, 2008 |
Cytokine receptors (cytoplasmic tyrosine kinases) pathway | TypeI: JAK→STATs→IRF→ISRE TypeII: JAK→STAT1→GAS Non‐canonical: JAK→ MAPK/PI3K/AKT | Inflammatory and immunological response, cell proliferation and haematopoiesis; regulated survival of injured neurons, neurite elongation, and re‐establishment of neuronal connections. | Miyajima, Kitamura, Nobuyuki, Takashi, & Kenichi 1992 O’Sullivan, Liongue, Lewis, Stephenson, & Ward, 2007 Valentino & Pierre, 2006 |
Autophagy pathway | mTOR→ ULK complex (ULK1, ULK2, mAtg13)→ FIP200→ Class III PI3K complex (hVps34, Beclin 1, p150, Atg14L) | Stress survival and longevity, elimination and recycling of damaged cellular components generated in response to induced oxidative stress or during normal aging, promoting constant cellular renewal, cell growth, development, and homeostasis. | Levine & Kroemer, 2008 Mizushima, Levine, Cuervo, & Klionsky, 2008 Yang & Klionsky, 2010 |
Apoptosis pathway | Bcl‐2 family (Bax, bak, Noxa, PUMA)→ cit. C, Smac/Diablo, Omi/HtrA2→ Caspase 9 & Efector Caspases Non‐canonical: TNF receptor Superfamily (CD95/Fas/Apo, TNF‐R1), Sphingomyelin‐Ceramide | Embryogenesis and the maintenance of tissue homeostasis during the stage adult, pathological conditions or in healthy tissue (for example, its participation in the development of nervous system and immune, or neurodegenerative dementias). | Almeida, 2012Brunelle & Letai, 2009 Gamen, Anel, Piñeiro, & Naval, 1998 Kolesnick & Golde, 1994 |
The Hippo pathway | DCHS½→FAT4→FRMD6/Mer/KIBRA→Mst½ →Mob1→Lats ½ →YAP/TAZ | Stem cell and progenitor self‐renewal, cell proliferation, antiapoptosis Organ Size control | Huang, Wu, Barrera, Matthews, & Pan, 2005 Zhao, Li, & Guan, 2010 Pan, 2010 |
Complement Cascade | Classical: IgG/IgM → C1q → C4/C2 → C3 →C5 Non‐canonical: Lectin: MBL → MASPs→ C4/C2 → C3 Alternative: C3‐H2O → Factor B/Factor D/Properdin → C5 | Immunoregulatory functions (enhancing humoral immunity, modifying T cell immunity, shaping the development of the natural Ab repertoire, regulating tolerance to nuclear self Ags such as DNA and chromatin). Pathogenic role (initiation and regulation of inflammatory response, opsonization and phagocytosis, systemic organ ischemia/reperfusion) and autoimmune diseases. | Alexander, Anderson, Barnum, Stevens, & Tenner., 2008Stevens et al., 2007 Thurman & Holers, 2006 |
Marijuán 2013. Reproduced with permission of Elsevier.
4.3.2 Counteracting the Donnan Effect: “Sensing the Solvent”
The Donnan (or Donnan‐Gibbs) effect refers to the osmotic disequilibrium that a living cell experiences due to the fact that it contains predominantly negative charges (i.e., nucleic and amino acids moieties) separated from the liquid environment by a semipermeable membrane. As a result of the opposing osmotic and ionic influences generated, a series of ionic and solvent exchanges follow with the net result that the membrane swells and finally bursts. To prevent this catastrophe, one of the earliest inventions of living cells was a series of molecular mechanisms actively counteracting the Donnan effect: stretch‐activated channels, voltage‐gated channels, ionic pumps (Na/K), aquaporins, etc. These mechanisms manipulate ionic/osmotic equilibria to restore the appropriate levels of mechanical stress, electrical potential, and ionic concentration gradients across the cell membrane.
Neurons are the great specialists in this ancestral toolkit, as usual with ample recombination of elements, using it to assemble an information processing system based on the generation and circulation of electrical perturbations along a network of membrane potentials. Ion channels associated with ligand receptor domains serve as fundamental portals for most neurotransmitters, particularly the fast‐acting ones (glutamate, acetylcholine, glycine, GABA). In addition, voltage‐gated channels of K+, Na+, Ca++, and Cl− classes integrate and propagate electrical perturbations of the membrane potential across neurites and cell bodies. The kinetic response properties of each channel and its state transitions—as well as those of any transient “inactive” states—are carefully fine‐tuned by means of differential splicing, so that dozens and dozens of slightly different types are deployed as needed for the different functions. For instance, there are over 80 mammalian genes that encode potassium channel subunits (Alberts et al., 2002), each subject to post‐transcriptional and functional modifications, to provide channel types specific to each intraneuronal microdomain. This exquisite control over electrical dynamics along the neuron makes possible sophisticated computations, but is extremely energetically expensive. The well‐known Na/K pump, part of the prokaryotic inheritance, is a key element within the osmotic/ionic toolkit maintaining the membrane potential of neurons—and represents the highest metabolic expense of the nervous system, up to 2/3 of the total energy budget of the brain (Alberts et al., 2002).
The fast changes in membrane potential governed by these pathways support cellular excitability in neuronal, muscular, renal, cardiac and epithelial tissues. However, it is not only cellular excitability that emerges from the osmotic toolkit—a new form of sensory detection has also been derived from the osmotic sensors of prokaryotes. The mechanosensitive (MS) ion channels initially acted as “emergency valves” in primitive prokaryotic cells, but evolved in multicellulars (animal, fungal, and plant) into an extraordinary range of mechanosensors and transient receptor proteins (TRPs) detecting gravity, growth, blood pressure, muscle tension, sound, thirst, heat, and so on (Kung, Saimi, & Martinac, 1990; Kung, 2005)
In point of fact, the receptors of sensory neurons can be broadly classified as either derived from toolkits for solute detection (vision, smell, taste, hormones, pheromones, nutrients, neurotransmitters, neuropeptides) or solvent detection (sound, touch, pressure, texture, proprioception, osmolarity, heat, volume, vibration) (Kung et al., 1990). In the first case, receptors associated with G protein pathways are the most frequent sensory detection mechanisms, while in the second case, mechanosensitive channels and TRPs become the central tools. Mechanical channel‐based senses differ from other senses in the close association of their receptors with the membrane lipids, explaining their higher susceptibility to anesthetics, and with the cytoskeleton, explaining their ability to transduce forces acting on cellular structures into electrical currents.
Therefore, in the same way that a number of eukaryotic receptors and pathways derive from the solute detection systems of prokaryotes (the “1‐2‐3” scheme), many eukaryotic sensory systems, notably the MS channels and TRP subfamilies (TRPV, TRPC, TRPA, TRPP, etc.), derive from homologous MS channels of bacteria and archaea used in solvent detection (Kung, 2005). The impact of these ancient mechanisms is impressive: Most of the electrical signaling toolkit used in neural computations is directly derived from the osmotic toolkit of prokaryotes.
4.3.3 The Interface between Signaling and Cell‐Cycle Control
Discussing the role of the prokaryotic cell cycle in signaling demands a change of perspective. Unlike the two previously discussed roots of eukaryotic signaling systems, the cell‐cycle machinery cannot be considered as a mere subcomponent or toolkit for neural computation. Rather, the cell cycle acts as the main “consumer” of the intracellular signaling system and as the cellular “chief executive”—it is central in the eukaryotic signaling network, linking sensory signaling pathways to powerful effector systems. The cell‐cycle machinery inherited from prokaryotes seems to have projected itself or erupted towards the cell surface, accessing relevant guidance cues from the external environment and, in so doing, to have subordinated many previously independent pathways for solute‐ and solvent‐detection.
The most powerful set of protein kinases in the signaling network is directly associated with mitotic control: the MAPK cascade (MAPKKK, MAPKK, MAPK). Depending on the cellular context, this cascade can be divided into three branches: MAPK/ERK, SAPK/JNK, p38/MAPK. Whatever receptors and transducers happen to be associated with the successive kinase hierarchies of these cascades come to occupy a highly‐privileged position in the control of the cell cycle and the life and death decisions of a neuron–and these associations are quite flexible, involving a variety of inputs (see Figure 4.2.). For example, one of the functions attributed to MAPK cascades is to integrate information by crosslinking signaling pathways at appropriate levels of amplification within the kinase hierarchy.

Figure 4.2 Cell‐cycle Control.
Transitions between the different phases of the cell cycle (G1, gap; S, synthesis; G2, interphase gap; M, mitosis) are tightly controlled. The modular organization of the multicellular organism allows space‐time separation between cell cycle phases, mediated by a number of controlling signaling pathways. The signaling control is ultimately based on a cloud of internal and external signals, usually of opposed signs (activators vs. inhibitors), that carefully regulate the reproductive and specialization trajectories of cells and tissues. In the figure, activating signaling pathways promoting cell‐cycle progress bear the sign, while the inhibiting ones bear the
sign. The sign
denotes cellular checkpoints, which can result in progress or arrest depending on incoming factors. The signaling pathways associated with MAP kinases appear in italics.
Marijuán 2013. Reproduced with permission of Elsevier.
Control of the different phases of the cell cycle (G1, S, G2, M) and their respective transitions is a crucial piece of the modular organization of multicellular organisms. Such population control is ultimately based on a cloud of internal and external signals, usually of opposed signs (activators vs. inhibitors), which carefully regulate the reproduction and differentiation of cells and tissues. These interactions require the cell cycle control system to “erupt” toward the cellular surface in metazoa. Most of the signaling pathways listed in Table 4.1 (§4.4.2) share information with cyclins, MAPK cascades, phosphatases, checkpoints, and other protein complexes involved in the cell‐cycle control. Throughout these pathways, multiple growth factors and apoptotic factors are secreted locally from neighboring cells, from more distant paracrine sites, and through interactions with the extracellular matrix. The balance between growth factors and apoptotic factors determines the developmental patterning of the multicellular organism by propelling cellular growth, eliminating transformed, senescent, or redundant cells, and generally keeping organs and tissues within functional bounds—processes which are especially crucial (and complex) in neural synapses, which behave much like autonomous cellular subunits. Thus, the elimination of decaying synapses, as well their maintenance, growth, and motion along the dendritic arbor, is strongly related to the balance between signaling pathways, including cell‐cycle control mechanisms, at the postsynaptic site. These mechanisms play a crucial role in synaptic plasticity and thus in learning, usually by activating or inhibiting synaptic development based on the relative pattern of electrical activity between the dendrite and axon of the postsynaptic neuron.
As a reminder of the symbiotic origins of eukaryotes, the cell‐cycle control network mediating irreversible apoptosis is localized to mitochondria—specifically, to the Bcl‐2 protein linked with the integrity of the respiratory chain. This makes a great deal of evolutionary sense, involving mitochondrial compatibility with the host cell as a form of selection for cells and organism with functional respiration (Blackstone & Green, 1999; Lane, 2011). The metabolic centrality of mitochondria makes them an important target for a number of signaling pathways, a crossroads where metabolic state, cell‐cycle state, and external signals convene into fundamental “checkpoints” deciding cellular fate. Notwithstanding their metabolic and cellular importance, mitochondria do not directly signal to other cellular subsystems. The surrender of almost all mitochondrial genes to the cell nucleus genome has deprived mitochondria of their own independent downstream signaling—even in the apoptotic case just mentioned, key proteins belong to the host cell.
4.3.4 The Cytoskeleton and Endocytic Matrix: Signaling Incorporation of Mechanical and Membrane‐Remodeling Systems
A dynamic cytoskeleton and a dynamic membrane are primordial traits of eukaryotes. Probably they coevolved, as they are functionally interdependent—and presumably both derived from the phagocytic life style of the LECA and early eukaryotes. With their increasing complexity, these two cellular systems have represented important mechanisms for both upstream and downstream extension of the primordial signaling pathways discussed above.
4.3.4.1 Endocytosis and the vesicular trafficking of receptors and messengers.
Endocytosis is one of the cellular processes most tightly associated with signaling dynamics; together they might be conceptualized as a single process central to the cellular “master plan” of the eukaryote (“two sides of the same coin”, according to Scita & Di Fiore, 2010). The integration of signaling molecules into the vesicle dynamics of membranes is crucial. In a number of pathways, recycling of receptors to and from the plasma membrane by means of endocytic/exocytic cycles serves to regulate receptor density and thus signal sensitivity; AMPA and NMDA glutamate receptors are among the best known instances (§4.5.2). Besides the recycling of receptors, the differentiation of endocytic routes serves as an additional way of regulating signaling activity. For instance, clathrin‐mediated endocytosis targets receptors for recycling and signaling continuance, while non‐clathrin‐mediated endocytosis targets them for degradation (Mills, 2007; Scita & Di Fiore, 2010).
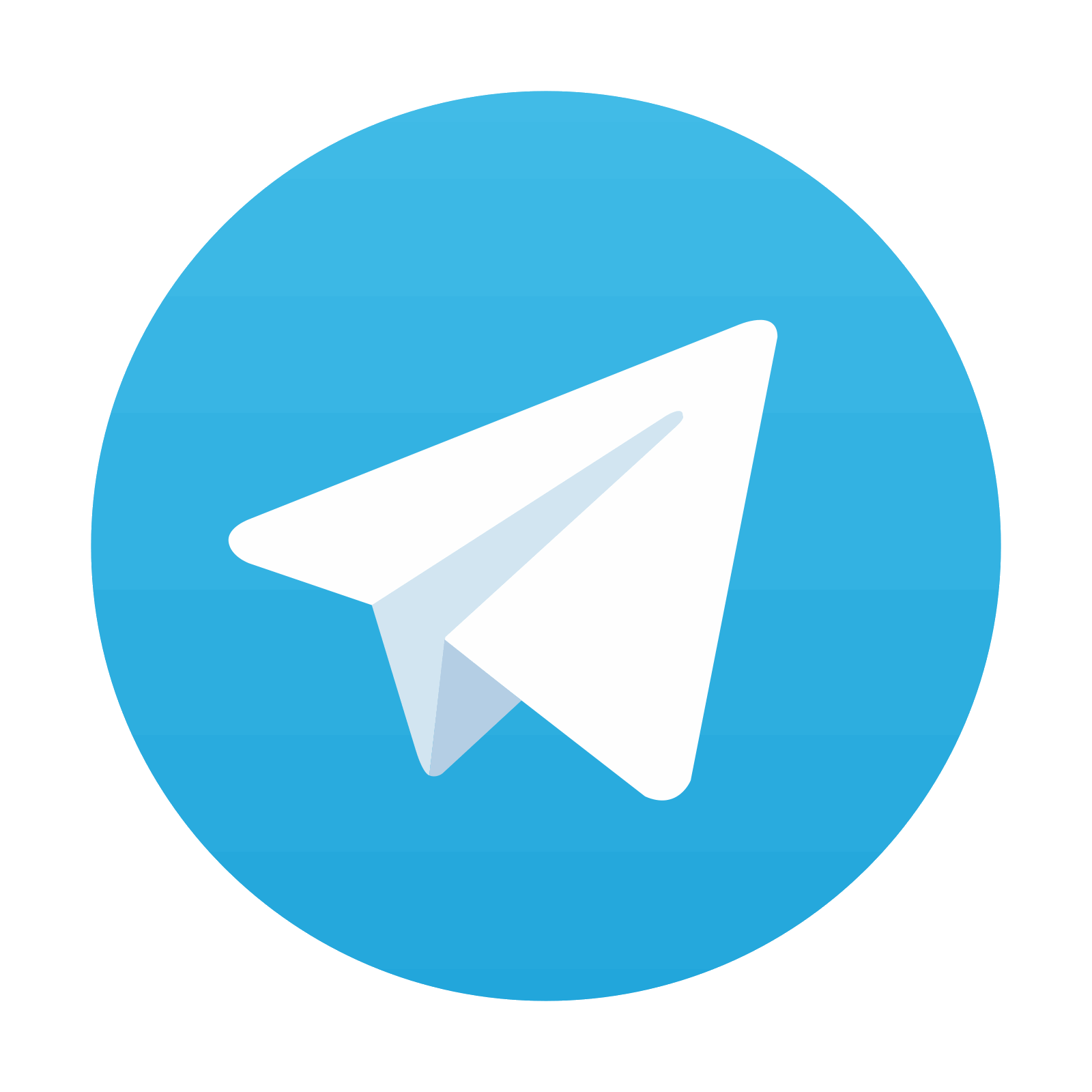
Stay updated, free articles. Join our Telegram channel
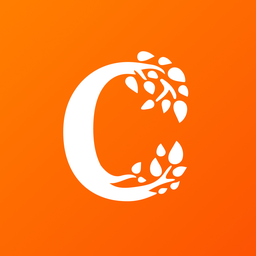
Full access? Get Clinical Tree
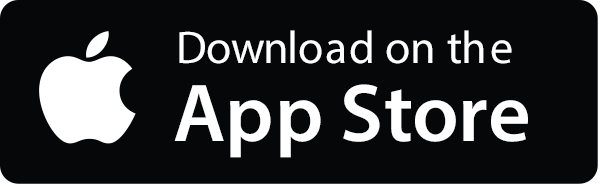
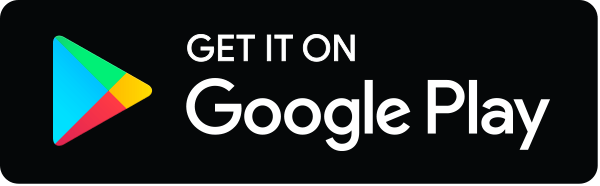