Over the past three decades, the field of intraoperative neurophysiologic monitoring has evolved and matured, and monitoring during procedures such as resection of acoustic nerve tumors and correction of spinal deformities is now considered the standard of care. Intraoperative monitoring can diminish the risk of neurologic injury during surgery in three ways. Nearly real-time functional surveillance of neural structures may enable detection of injury at a time when it can be reversed or minimized. For example, during surgery for correction of spinal deformities, monitoring by somatosensory evoked potential (SEP) and motor evoked potential (MEP) recordings can detect deteriorating long-tract function early enough to avert permanent spinal cord damage. Intraoperative neurophysiologic techniques can also be used to identify neural structures that are difficult to locate visually. SEPs, for example, are used commonly to identify the rolandic fissure during resective cerebral surgery. Finally, monitoring occasionally provides insights into the pathophysiology of intraoperative neurologic injury that may lead to improvements in surgical practice, anesthetic management, and neurophysiologic technique.
The development of effective intraoperative neurophysiologic monitoring strategies has resulted from the combined efforts of neurophysiologists, anesthesiologists, and surgeons. For the neurophysiologist, transposition of neurophysiologic recording techniques from the traditional diagnostic laboratory setting to the operating room has entailed the modification of standard recording techniques as well as the development of new ones. It has also been necessary to develop interpretative strategies appropriate for the operating room. For the anesthesiologist, this has meant adaptation of anesthetic techniques to facilitate neurophysiologic monitoring. For the surgeon, optimal utilization of intraoperative monitoring has required learning the appropriate questions to ask the neurophysiologist and what to do with the answers.
Careful examination of early reports of failures of evoked potential (EP) monitoring to detect neurologic injury reveals that some of these failures did not reflect limitations inherent to EP monitoring per se, but rather were the consequence of errors such as the failure to monitor for a sufficient time, failure to monitor the correct type of EP, and failure to recognize artifact. The occurrence of these types of failures reflects the learning curve that is an inevitable part of the development of a new family of technical procedures. The field of intraoperative monitoring continues to evolve, and in some cases there are significant differences in the techniques used at various centers. The current literature contains an unending stream of papers describing innovative, imaginative, and often effective approaches to various aspects of intraoperative monitoring. This chapter is not intended to serve as either an exhaustive reference or an instruction manual. Rather, it is intended to provide an introduction to the major clinical areas in which intraoperative monitoring is used currently and to illustrate how standard diagnostic laboratory techniques may be extended for use in the operating room.
Long-tract monitoring
One important application of intraoperative monitoring is the surveillance of long-tract function during procedures that place motor and sensory tracts at risk. These include certain orthopedic spinal procedures, invasive neuroradiologic procedures, spinal cord and brainstem surgery, and surgery involving the abdominal aorta. Historically, the “wake-up” test was used to verify the functional integrity of the spinal cord during certain spinal procedures. However, the wake-up test provides only a single, nonquantitative indication of spinal cord function, and it exposes the patient to additional risks including dislodgment of instrumentation, laminar fractures, pulmonary embolism, and accidental extubation. When the wake-up test is performed, its typical role is now confirmatory, rather than one of primary surveillance.
Because SEPs and MEPs are mediated by anatomically segregated pathways, independent intraoperative injury can occur to either pathway. During surgery for intramedullary lesions, SEPs may be lost due to posterior column disturbance at the time of the initial myelotomy when there has been no injury to motor tracts, precluding further SEP monitoring. The vascular anatomy of the spinal cord, with anterior and lateral portions being dependent on the anterior spinal artery and the posterior columns supplied independently and more robustly by the posterior spinal arteries, provides a further basis for independent injury. The anterior spinal artery has large watershed regions along its length, making the corticospinal tracts particularly vulnerable to ischemia resulting from hypotension and potentially producing loss of motor function without altering SEPs. During scoliosis surgery, distraction or derotation may cause occlusion or spasm of the artery of Adamkiewicz, the major radicular branch supplying the lower portion of the anterior spinal artery. This can disrupt the blood supply to the corticospinal tracts, with preservation of posterior column perfusion leaving SEPs intact. For similar reasons, MEP monitoring is employed during thoracic abdominal aneurysm repair at some centers where it can have important input into real-time management decisions. These decisions include discontinuation of partial bypass, the selection of segmental arteries for reimplantation, and changes to blood pressure, cardiac output, and bypass flow rate. Independent loss of MEPs and SEPs may be observed during provocative testing with intra-arterial lidocaine injection before endovascular embolization of spinal cord vascular malformations; the modality lost following injection of anterior or posterior circulation may be unpredictable because of abnormal angioarchitecture.
In general, SEP and MEP monitoring are complementary, and optimal monitoring of long-tract function often entails concurrent recording of both SEPs and MEPs. Despite their dependence on distinct anatomic pathways, each modality provides good surveillance of spinal cord integrity in many cases. Experimental spinal cord injury caused by ischemia, compression, and blunt trauma produces graded changes of both MEPs and SEPs. Operative injury, particularly injury caused by spinal cord compression, generally produces changes in both MEPs and SEPs. Importantly, it is possible that either measure may be compromised by electrical interference or other factors that can make its interpretation difficult or ambiguous; for this reason, concurrent MEP and SEP recording can provide an added level of security. Additionally, concomitant monitoring of both upper and lower extremities can provide a basis for distinction of systemic or anesthetic effects from local surgical injury.
SEP Monitoring
Although the techniques used for intraoperative SEP recording are similar to those used in the diagnostic laboratory, important differences exist. Among these are the effects of certain anesthetic agents that can attenuate the cortical components of SEPs, anesthetic agents that may augment intraoperative cortical recordings, and the use of paralytic drugs that can actually facilitate the recording of subcortical SEP components.
The montages used to record SEPs, both in the diagnostic laboratory and in the operating room, exploit the restricted scalp topographies of the primary cortical SEP components and the widespread distribution of the far-field subcortical components. For the median nerve SEP, the primary cortical N20 response is confined to the centroparietal portion of the scalp opposite the stimulated arm, whereas the subcortical P14 and N18 responses are widely distributed. Accordingly, a bipolar “scalp-to-scalp” recording between symmetric centroparietal scalp electrodes on either side of the head detects the N20 response in isolation. By contrast, a referential “scalp-to-noncephalic” recording, which uses a scalp electrode ipsilateral to the stimulated median nerve, detects only subcortical far-field potentials ( Fig. 30-1 ). The topography of posterior tibial nerve SEPs is somewhat more complex, and varies considerably among individuals. The primary cortical P37 response may be largest at the vertex or over the scalp ipsilateral to the stimulated leg. For this reason, it is necessary to use at least two channels to record P37 reliably in all patients (e.g., CPz–CP contralateral as well as CP ipsilateral–CP contralateral), or to determine the optimal derivation for recording P37 by mapping the response prior to monitoring. (Electrode locations CP ipsilateral and CP contralateral refer to standard CP3 and CP4 locations, respectively ipsilateral and contralateral to the stimulated limb.) The subcortical P31 and N34 components are detected in isolation by using a referential Fpz electrode to a noncephalic derivation.

In the diagnostic laboratory, N20 and P37 cortical responses are typically easy to record, even in awake patients. The relatively short interelectrode distances minimize movement- and muscle-related artifacts. However, in the operating room, cortical SEP components can be difficult to record reliably because they are attenuated by most inhaled general anesthetic agents. This can cause cortical SEP components to be unstable and change in both amplitude and latency with variations in anesthetic drug concentration. These effects are greatest in infants and young children and are generally more prominent for lower-extremity SEP recordings. Both halogenated inhalational agents (e.g., halothane, isoflurane, sevoflurane, and desflurane) and nitrous oxide reduce cortical SEP amplitudes and prolong SEP latencies. It appears that the combination of halogenated anesthetics with nitrous oxide has more profound effects on SEP latency and amplitude than equipotent doses of these anesthetic agents used alone. Likewise, because sevoflurane, desflurane, and nitrous oxide are insoluble relative to halothane and isoflurane, anesthetic effects on the SEP may vary rapidly as delivered concentrations are changed intraoperatively. Most other agents (e.g., propofol, dexmedetomidine, opioids, benzodiazepines, and barbiturates) have similar but considerably less prominent effects.
In the diagnostic laboratory, subcortical far-field potentials are often difficult to record, largely due to the susceptibility of noncephalic referential recordings to contamination by movement- and muscle-related artifacts. In the operating room, muscle-related noise can be eliminated, and the recording of subcortical signals correspondingly facilitated through the use of neuromuscular blocking agents ( Fig. 30-2 ). Subcortical SEP components are much less affected by anesthetic agents, and for this reason can be particularly useful indicators for intraoperative monitoring ( Fig. 30-3 ).


Subcortical signal can be occasionally difficult to record, particularly in patients with pre-existing neurologic deficits. Further, in cases where concurrent MEP or electromyographic (EMG) monitoring limits or precludes the use of neuromuscular blockade, it is particularly important to be able to monitor cortical responses. In these cases, agents that have less effect on cortical SEPs (e.g., propofol or dexmedetomidine) are desirable, and those that most attenuate the cortical SEPs (e.g., halogenated inhalational agents) should be limited. Communication with the anesthesiologist is essential because small variations in the doses of anesthetic drugs, particularly halogenated inhalational agents and nitrous oxide, may produce large variations in SEP amplitude that can mimic the effects of surgical injury. Cortical SEP amplitudes may exhibit similar sensitivity to fluctuations in blood pressure ( Fig. 30-4 ). Additionally, etomidate, a gamma-aminobutyric acid (GABA) A receptor agonist, and ketamine, an antagonist of N -methyl- D -aspartate (NMDA) receptors—intravenous anesthetic agents that have been shown to increase the amplitude of cortical SEP components—can be used to augment cortical SEPs intraoperatively. Figure 30-5 presents an example of SEP monitoring using subcortical and cortical SEPs and the use of etomidate to enhance the cortical signals.


Surgical injury to the spinal cord typically produces loss of EP amplitude and degradation of signal morphology; latency prolongations are a less prominent and consistent finding. Interpretive strategies must therefore be different from those used in standard laboratory SEP testing, which are based primarily on response latencies. Moreover, for intraoperative monitoring, a patient’s EPs are compared primarily with that patient’s baseline values rather than normative controls.
Two somewhat different approaches have been used to interpret intraoperative SEP recordings. One is to adopt predefined limits beyond which the risk of neurologic insult is considered to be substantial and to inform the surgeon when those limits have been reached. Many centers, somewhat arbitrarily, use a 50 percent decrement in amplitude or a 10 percent increase in latency for these limits. An alternative approach is to inform the surgeon of changes, even if small, in SEP amplitude, latency, and morphology that are determined by the neurophysiologist to have exceeded the baseline variability in that patient’s recordings. The authors favor the latter approach because it better enables the surgeon to identify the cause of SEP changes and to use that information to decide whether to take a “wait-and-see” approach or to act immediately.
The implication of SEP deterioration is very much dependent on the surgery being performed. The loss of SEP amplitude during correction of spinal deformities is ominous and carries with it a high risk of serious neurologic injury in the absence of corrective measures. By contrast, the loss of SEP amplitude during intramedullary surgery is often predictive of immediate postoperative neurologic deficits but is less often predictive of the eventual neurologic outcome. Surgical manipulation of the spinal cord can cause transient conduction block without permanent axonal injury. Further, while the stability of SEPs during intramedullary surgery can provide useful reassurance to the surgeon that neurologic injury has not occurred, the loss of SEPs tends to be abrupt and not serve as a warning of impending injury.
MEP Monitoring
The integrity of descending motor pathways can be monitored intraoperatively by recording the signals from spinal cord or muscle that are evoked by transcranial stimulation. Transcranial stimulation may directly fire pyramidal neurons or may activate cortical interneurons, which then fire pyramidal cells indirectly. Intervening synapses prevent retrograde firing of sensory tracts. Direct pyramidal stimulation produces the earlier, more stable components of the MEP known as D waves, whereas indirect pyramidal activation via interneurons results in the longer-latency, less stable I waves ( Fig. 30-6 ). D waves are relatively resistant to attenuation by anesthetic drugs, including volatile anesthetics, while I waves are highly susceptible. In general, the temporal summation of a series of descending volleys, either D wave followed by subsequent I wave or a series of closely spaced D waves, is required to activate spinal motor neurons.

While motor pathways may be activated by both electrical and magnetic stimulation, transcranial magnetic MEPs are attenuated substantially by most commonly used anesthetics, and are not sufficiently reliable for intraoperative monitoring. Instead, intraoperative MEP monitoring relies on transcranial electrical stimulation. Transcranial electrical MEPs are elicited using anodal stimulation. For lower-extremity stimulation, the anode may be placed at Cz, with the cathode at C1 or C2, C3 or C4, or Fz, or utilizing linked electrodes around the cranial base. Alternatively, a single pair of electrodes may be used at C1 and C2 or C3 and C4, reversing electrode polarity to produce greatest-amplitude compound muscle action potentials (CMAPs) contralateral to the anode. Lower stimulation intensity generally is required to elicit MEPs from the upper extremities than from the lower; greater intensities are also likely to produce deeper effective stimulation, activating the corticospinal tracts at the level of the internal capsule. MEPs may be elicited using either special purpose capacitive-coupled constant-voltage stimulators, or standard constant-current SEP-type stimulators. The former provide more rapid delivery of charges: 1 coulomb/sec compared to 0.1 coulomb/sec. Both types of stimulator provide satisfactory stimulation for MEP monitoring, although the capacitive-coupled constant-voltage type has been found to require lower total charge delivery.
The effectiveness of transcranial electrical stimulation is enhanced considerably by using a series of rapidly delivered pulses rather than a single pulse. This likely results from temporal summation at both cortical and spinal levels. In one study, prominent increases in CMAP amplitude and decreases in latency occurred as the interpulse interval decreased from 10 msec to 1 msec ( Fig. 30-7 ). In another study, simultaneous recordings from epidural space and muscle demonstrated only D waves and no muscle response to a single pulse, or to two transcranial pulses 2 msec apart. However, there were D waves, multiple I waves, and a CMAP to trains of three or four pulses. In patients with no pre-existing motor deficits undergoing various neurosurgical procedures under total intravenous anesthesia without neuromuscular blockade, the lowest motor thresholds were achieved with a train of five monophasic constant-current 0.5-msec pulses with an interstimulus interval of 4 msec. Dual train stimulation, in which the test stimulus train is preceded by a conditioning train that heightens motor neuron excitability, can be used to enhance MEPs further. Journee described a trimodal facilitation curve with optimal response at intertrain intervals of approximately 10 and 20 msec, and again at greater than approximately 150 msec. Proper selection of intertrain interval appears to be critical; intertrain intervals of 30 to 150 msec produced attenuation, rather than facilitation, of the response to the second stimulus train ( Fig. 30-8 ).


MEPs may be monitored by recording neural activity in the epidural space, subdural space, or peripheral nerve, or CMAPs from target muscles. During 160 surgeries for intramedullary lesions, Kothbauer and associates reported success in recording epidural MEPS in about two-thirds of cases. With adequate stimulation (i.e., 2 to 3 times threshold), epidural D waves to transcranial stimulation are robust and relatively stable. Unchanged epidural MEPs were reported to be associated universally with preserved function, whereas reduction of epidural MEPs over 50 percent generally was associated with postoperative deficit. Epidural recording has the advantage of reduced sensitivity to anesthetic agents, which depress spinal motor neurons as well as cortical activity. It is compatible with the use of volatile anesthetics as well as complete neuromuscular blockade. The amplitude of epidural D waves, however, is greatest rostrally, and diminishes substantially below T8. Further, D-wave monitoring cannot detect spinal motor neuron ischemia. Epidural recordings therefore are not well suited to detecting lesions affecting lower spinal cord, cauda equina, or spinal roots, and cases in which detection of unilateral lesions is an important consideration. During scoliosis surgery, spurious decreases, as well as increases in D-wave amplitude, have been reported, likely resulting from change in position of spinal cord relative to the epidural recording electrode during correction.
CMAP recordings, by contrast, allow the lower spinal cord, cauda equina, and spinal roots to be monitored, and are well suited to detection of unilateral lesions. For spinal cord monitoring, the most reliable responses are often obtained from distal muscles, in particular abductor pollicis brevis (APB) and abductor digiti minimi (ADM) for the upper extremities and abductor hallucis (AH) for the lower extremities, perhaps reflecting their higher density of corticospinal tract innervation. For this reason, assuming that these muscles are functionally intact at baseline, they generally should be included in MEP monitoring of spinal cord function. CMAP recordings are sensitive to commonly used inhalational anesthetics, and total intravenous anesthetic techniques are preferred in some centers. Anesthetic techniques that include a continuous infusion of propofol, a GABA A receptor agonist, or dexmedetomidine, a selective central alpha 2 receptor agonist, combined with an opioid (e.g., fentanyl, sufentanil, or remifentanil) and occasionally supplemented by low-dose ketamine, are compatible with CMAP recording. However, by employing high-output (500 V) multipulse stimulators, CMAP MEP monitoring can often be compatible with the use of either nitrous oxide or isoflurane anesthesia, or both. Partial neuromuscular blockade, produced by an infusion of nondepolarizing muscle relaxant, may be used to reduce movements that would interfere with surgery and EMG activity that compromises SEP monitoring, yet still permit reliable monitoring of CMAP MEPs. Figure 30-9 illustrates concurrent SEP and MEP recordings during surgery for correction of a spinal deformity in which there was unilateral loss of both SEPs and MEPs, with subsequent partial recovery of the SEP. Postoperative examination revealed a corresponding monoparesis.

Examination of adverse events in over 15,000 published and unpublished instances of patients monitored by transcranial electrical MEPs reveals a favorable safety profile. There is no apparent association of transcranial electrical MEPs with adverse cognitive, affective, or endocrine effects. The most common adverse events were bite injuries (29 patients) to lips or tongue, and a single mandibular fracture. Additional associated adverse events included cardiac arrhythmias (5 patients), seizures (5 patients), scalp burns (2 patients), and intraoperative awareness (1 patient). The occurrence of bite-related injury has prompted the suggestion that soft bite-blocks, such as rolled gauze, be used. The rare arrhythmias and seizures may have been coincidental, although the authors have encountered one (additional) case of bradycardia that was associated reproducibly with transcranial electrical stimulation. This occurred in a 65-year-old patient with history of cardiac disease; bradycardia resolved promptly upon cessation of stimulation, and there were no sequelae.
Interpretive Criteria
Unlike SEP recording, in which all stimuli produce identical responses, there is considerable within-patient, trial-to-trial variability of CMAPs elicited by both transcranial and direct spinal stimulation ( Fig. 30-10 ). This complicates the establishment of clear criteria for abnormality. Various criteria have been proposed, ranging from amplitude reduction to 50 percent or 20 percent of baseline, to complete loss of MEPs in consecutive trials. Criteria based on MEP waveform morphology and stimulus threshold intensity have also been suggested.

As with SEP monitoring, an alternative approach is to inform the surgeon of changes in MEP response (amplitude, latency, and morphology) that exceed the baseline variability in the patient’s recordings, realizing that the reproducibility of MEPs may be influenced by various factors, including anesthetic technique and stimulation protocol. Although this approach is somewhat subjective because the criteria employed are quantified less easily, we currently favor its use. It avoids application of arbitrary and simplistic criteria, and also enables the surgeon to best identify causes of MEP changes. For monitoring of thoracolumbar cord function, correlation with MEPs recorded from thenar muscles is useful for identifying confounding systemic effects.
Cranial nerve and spinal root monitoring
Intraoperative electrophysiologic monitoring provides a means of identifying cranial nerves and spinal roots and allows continuous monitoring of their functional integrity. The facial nerve commonly is monitored during acoustic neuroma and other cerebellopontine angle surgery, where it is at risk for being injured or inadvertently severed. Particularly with large tumors, cranial nerve VII may be difficult to identify visually if it is spread apart by or enmeshed in the tumor or tumor capsule. Several early studies have confirmed the efficacy of facial nerve monitoring for reducing morbidity following acoustic neuroma surgery. In a comparison of 91 consecutive monitored cases with 91 control subjects matched for age, tumor size, and year of surgery at the Mayo Clinic, 45 percent of the monitored but only 27 percent of the unmonitored patients were free from deficit. Two percent of the monitored and 6 percent of the unmonitored patients had no facial nerve function.
Additionally, cranial nerves III, IV, and VI may be monitored during surgery involving the cavernous sinus. Lower cranial nerves are monitored during skull base surgery, including resection of clivus tumors and tumors of the jugular foramen. Spinal roots are monitored during procedures such as tumor resection, spinal cord untethering, and placement of pedicle screws, all of which carry the risk of injury.
Triggered EMG
When cranial nerves or spinal roots are difficult to identify visually because of abnormalities such as scarring or anatomic distortion, they can be identified electrophysiologically by electrically stimulating the tissue in question and recording CMAPs over appropriate muscles. In contrast to transcutaneous stimulation, where constant-current stimulators generally are used, constant-voltage stimulators are often preferred for stimulation within the surgical site. Constant-current stimulators are favored in the standard laboratory setting because they deliver relatively constant depolarizing current to the nerve despite variations in electrode impedance. In the operative field, however, current shunting by ambient fluid, rather than contact impedance, is the major concern. Constant-current stimulators can deliver widely varying currents to a nerve, depending on whether the field is dry or covered by cerebrospinal fluid; a more uniform current actually is delivered to the nerve by a constant-voltage stimulator.
Either surface or intramuscular electrodes are suitable for recording CMAPs following electrical nerve stimulation. If needed, partial neuromuscular blockade may be used to suppress movement, but typically is avoided altogether. CMAPs recorded from direct nerve stimulation are compatible with the use of either inhalational or intravenous anesthetic techniques. Stimulation is performed with a hand-held monopolar probe controlled by the surgeon. Initial “searching” for nerve roots is performed at relatively high stimulus intensities. The stimulus intensity is then decreased so that the target root is stimulated selectively to confirm its identity and establish its stimulation threshold. The stimulation threshold is about 0.03 to 0.1 mA for healthy bare cranial nerves and slightly higher for roots. Throughout dissection, the nerves are probed repeatedly. When the immediate objective is to confirm that the area to be resected or cauterized does not contain nerve, high stimulation intensities (about three times threshold) are used. To confirm the identity of the nerve, that nerve’s threshold intensity is used. It is important to note that, besides lack of proximity to the nerve, failure to stimulate can reflect nerve injury causing conduction block or previous transection of the nerve proximal to the site of stimulation. The ease with which a nerve is stimulated may be predictive of postoperative function.
The proximity of muscles on the face can result in “crosstalk” between recording electrodes. Electrodes in muscles innervated by cranial nerve VII, for example, may also record signals generated by the masseter or temporalis muscles, which are innervated by cranial nerve V. Onset latencies of the CMAP can help to distinguish these responses. When stimulated intracranially, cranial nerve VII has a latency of about 6 msec, whereas cranial nerve V (motor) has a latency of about 3 msec. Stimulation of cranial nerve VI activates the lateral rectus muscle. This activity can be picked up in the “orbicularis oculi channel,” but at a latency of about 2 msec ( Fig. 30-11 ).
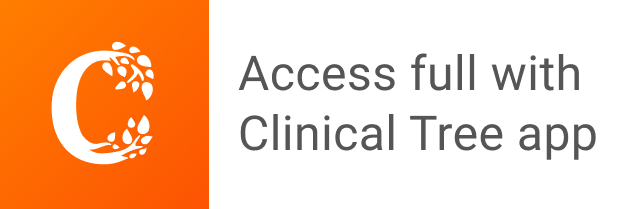