Abstract
Ischemic stroke is a leading cause of permanent disability worldwide. While intensive rehabilitation has the potential to improve motor function in some patients in the chronic phase of stroke, a large proportion of patients are left with significant motor deficits. Several noninvasive and invasive neuromodulatory approaches have been investigated as adjunctive therapies to augment motor recovery in the chronic phase. These modalities are aimed at modulation of the surviving perilesional cortex or remote cortical areas.
Noninvasive neuromodulatory strategies, including transcranial magnetic stimulation (TMS) and transcranial direct current stimulation (tDCS), have demonstrated the ability to produce significant improvements in motor function when combined with rehabilitation and are important investigational tools in elucidating mechanisms of recovery in human subjects. Invasive strategies that have been investigated include epidural cortical stimulation (ECS), vagal nerve stimulation (VNS), and deep brain stimulation (DBS). ECS of the motor cortex (MCS) demonstrated promise in preclinical studies using both rodent and nonhuman primate models of stroke. While Phase I and Phase II studies showed apparent benefits, a pivotal Phase III randomized controlled clinical trial (RCT) failed to demonstrate improvements when compared to rehabilitation alone. VNS has shown efficacy in recent studies and a Phase I clinical trial recently conducted in the United Kingdom demonstrated safety and promising benefits. Our group has investigated the use of DBS, targeting the dentatothalamocortical (DTC) pathway output. We have shown that DBS of the DTC augments motor recovery in rodent models of large and focal ischemic strokes. In addition, we have shown that DBS of the DTC increased expression of markers of synaptic plasticity in the perilesional cortex as well as synaptogenesis and significant reorganization of motor representation. A Phase I clinical trial of cerebellar DBS is currently underway.
While significant research efforts are still needed before neurostimulation becomes a standard treatment for poststroke rehabilitation, several investigational approaches have shown promising data.
Keywords
Deep brain stimulation, Epidural cortical stimulation, Neuromodulation, Neuroplasticity, Neurorehabilitation, Plasticity, Stroke, Vagal nerve stimulation
Outline
Introduction 1147
Proposed Mechanisms Underlying Functional Recovery 1147
Neurostimulation-Based Approaches to Enhance Motor Poststroke Motor Recovery 1149
Noninvasive Brain Stimulation 1149
Vagal Nerve Stimulation (VNS) 1149
Epidural Cortical Stimulation 1150
Deep Cerebellar Stimulation 1152
Future Trends and Pathways 1154
Conclusion 1155
References 1155
Introduction
Approximately 795,000 people suffer a new or recurrent stroke annually in the United States, with 87% of strokes caused by cerebral ischemia ( ). Ischemic stroke (IS) is a leading cause of disability worldwide, and indirect costs resulting from lost productivity due to stroke are projected to increase from $25 billion in 2010 to $44 billion in 2030 in the United States alone ( ). Although stroke survivors suffer from a wide array of neurologic impairments, upper extremity motor deficits are associated with the worst limitations for executing activities of daily living (ADLs), leaving patients dependent upon caregivers ( ). As stroke mortality has continued to decline and the number of individuals with poststroke disability has increased, the need for effective rehabilitation strategies and treatments to augment rehabilitation has grown significantly. In the current review, we will briefly summarize the leading mechanistic hypotheses regarding poststroke functional recovery and describe current neurostimulation-based approaches to facilitate or enhance those processes ( Fig. 95.1 ).

Proposed Mechanisms Underlying Functional Recovery
Following IS, most patients experience some degree of recovery of lost motor function. This period of spontaneous recovery typically ceases, however, 3–6 months after stroke, at which point functional disability plateaus and remains stable ( ). Robust evidence supports the use of rehabilitation to improve neurologic function even beyond the window of spontaneous recovery ( ). Nevertheless, a large number of patients remain chronically disabled and incapable of independently performing ADLs, underscoring the need for new technologies and interventions aimed at promoting poststroke motor recovery.
Poststroke neuroplasticity is believed to be a primary contributor to functional recovery. Following ischemic injury, surviving neural circuits are able to subsume the function of damaged cortical regions, a process termed vicariation of function. Although regions of the contralesional hemisphere are also thought to play a role in the recovery of function, most attention is paid to changes in the perilesional cortex surrounding the ischemic core ( ). Cortical reorganization manifests as changes in the motor representation map following ischemia and subsequent rehabilitation ( ). This process has been further linked to changes in cortical excitability ( ), with enhanced excitability at the neuronal level thought to facilitate the plastic changes that contribute to cortical reorganization and functional recovery. Indeed, such changes, including map size, have been shown to correlate with improved recovery following stroke ( ). One of the key goals of neurostimulation in poststroke rehabilitation is to promote or redirect plasticity that is mediated by activity. While electrical stimulation is expected to cause effects at the cellular and synaptic levels, discussed later, it is unlikely that it can drive rehabilitation of specific neurological functions (i.e., movement, speech) without pairing with activity-dependent rehabilitation strategies. In other words, the clinical goal of neuromodulation is not to replace physical, occupational, or speech therapy but, rather, to augment their effect.
At the cellular level, functional reorganization is accompanied by axonal sprouting, synaptogenesis, and, to a lesser extent, neurogenesis ( ). Studies in a variety of animal models have extensively documented the widespread occurrence of axonal sprouting in the peri-infarct cortex as well as at sites distal to the infarct ( ). The process of axonal sprouting is regulated by a number of factors. NogoA, EphrinA5, and chondroitin sulfate proteoglycans act to confine the growing axon, while prosprouting factors including GDF10, GAP43, and a variety of other cellular adhesion, axonal guidance, and cytoskeletal molecules encourage the sprouting and growth of axons after injury ( ). In addition to axonal sprouting, new synapses are formed as indicated by elevated expression of synaptophysin in the perilesional cortex in animals ( ). The processes of angiogenesis and neurogenesis occur in tandem within a neurovascular niche. Growth factors and chemokines secreted by angiogenic vessels further stimulate the migration of endogenous, neuronal, stem cells from the subventricular zone to the infarct core ( ). The neurogenic response, however, is limited in scope, and there is mixed evidence supporting its occurrence and functionality in humans.
At the synaptic level, long-term potentiation (LTP) plays an important role in poststroke recovery ( ). In the mid-20th century, Donald Hebb proposed that when a given neuron repeatedly or consistently activates another neuron, metabolic or morphologic changes occur such that the first cell’s efficiency in activating the second cell is increased ( ), hence, LTP. Today, an extensive body of literature supports the phenomenon of activity-dependent changes in synaptic strength. The postsynaptic, N-Methyl-D-aspartic acid (NMDA) glutamate receptor is critical for LTP induction, while intracellular CaMKII and protein kinase C (PKC) also have been shown to play a role ( ). The changes in synaptic strength induced by LTP are critical for cortical reorganization, whether in the process of normal motor skill learning or in the recovery of motor function after stroke.
Neurostimulation-Based Approaches to Enhance Motor Poststroke Motor Recovery
Noninvasive Brain Stimulation
Over the past several decades the prognostic and therapeutic value of noninvasive, neurostimulation techniques has been investigated. Transcranial magnetic stimulation (TMS) and transcranial direct current stimulation (tDCS) are the most widely used modalities for noninvasive brain stimulation (NIBS) ( ). TMS of the motor cortex was first used in poststroke patients to generate motor evoked potentials (MEPs) as a means of indexing the residual integrity of descending motor pathways ( ). TMS has also proven to be a valuable tool for mapping changes in the motor cortex during the course of recovery and rehabilitation ( ). Longitudinal investigations utilizing TMS have helped elucidate the role of cortical remapping and neuroplasticity in functional recovery. While these studies showed that TMS could provide important mechanistic and prognostic information with regards to potential motor recovery, further investigation also indicated that NIBS was capable of improving motor function in chronic stroke patients ( ). These studies provided some of the first evidence that modulation of the ipsilesional motor cortex could improve motor function in the chronic phase of stroke. There are, however, several barriers to widespread use of NIBS as a therapeutic modality. First, while improvements following NIBS may be statistically significant, they remain functionally modest, with sometimes limited impact on quality of life (QOL) ( ). Second, widespread adoption of TMS is limited by the need for serial outpatient visits involving large, expensive systems that typically are only available at select medical centers. The availability of tDCS stimulation systems may overcome the concern related to portability and repeated use. Difficulty in enrolling patients for clinical trials investigating NIBS has hindered the regulatory approval of noninvasive modalities, creating an additional barrier to access for patients ( ). Nevertheless, NIBS has provided key evidence supporting the potential of neurostimulation-based techniques to treat poststroke motor impairments and will remain an important tool in the evaluation and study of recovery. See Section XV for in-depth discussions of NIBS.
Vagal Nerve Stimulation (VNS)
Implantable neurostimulation devices allow for direct and chronic stimulation of the nervous system, overcoming some of the obstacles posed by NIBS. First investigated in the early 20th century as a tool to understand the anatomy and physiology of the nucleus tractus solitarius (NTS), vagal nerve stimulation (VNS) has since been investigated as a potential, treatment modality for a wide array of neurologic and nonneurologic diseases. Following preclinical studies of epilepsy, VNS was found to be an effective tool in suppressing seizures in patients with certain medically refractory, epilepsy syndromes ( ). The US Food and Drug Administration (FDA) granted this therapy approval for the treatment of partial-onset epilepsy in 1997. VNS has also been investigated as a treatment for a wide range of disorders including depression, migraine, movement disorders, neuropsychiatric diseases, inflammatory diseases and other forms of epilepsy ( ). For an in-depth discussion of VNS see Chapter 18Chapter 18Chapter 81 and for an in-depth discussion of vagal nerve stimulation for the management of disease, see Section XIV, edited by Christopher Czura.
The afferent fibers of the vagus nerve terminate predominantly in the NTS, which projects, in turn, to multiple brain regions, including other brainstem nuclei (dorsal raphe and locus coeruleus), the cerebral cortices, cerebellum, and spinal cord ( ). Preclinical studies of VNS have identified several physiologic effects of VNS including modulation of cerebral blood flow, neuroinflammation, excitotoxicity, and neurotrophic signaling ( ). VNS applied only during critical periods of activity is also capable of enhancing plasticity and reorganization in the sensory and motor cortices ( ). Given the overlap between the pathophysiologic mechanisms of IS and the therapeutic mechanisms of VNS, interest developed in the potential of VNS as a therapy for IS.
Initial preclinical studies were carried out in a rodent model of IS ( ). Rats were trained on an automated, behavioral task that involved reaching through a slot to depress a lever. Once proficient, ischemia was induced by injection of the vasoconstrictive agent, endothelin-1 (ET-1), into the motor cortex. After stroke, animals either received rehabilitation alone, or rehabilitation with VNS delivered in trains of 500 ms within 70 ms after initiation of the lever press. The authors found that rehabilitation combined with triggered VNS resulted in a return to prestroke performance levels while the rehabilitation only group demonstrated a persistent deficit after 5 weeks of treatment. A follow-up study using an isometric pull task showed that VNS therapy also enhanced restoration of forelimb strength and reaching performance ( ). Additional studies have also shown efficacy of VNS in improving motor function following IS in aged rats as well as a rat model of hemorrhagic stroke induced by striatal injection of collagenase ( ).
Based on these preclinical findings, a randomized controlled clinical trial (RCT) was recently carried out in the United Kingdom ( ). The study enrolled patients with a history of IS occurring at least 6 months prior to the study with moderate to severe arm impairment. Patients were randomized to receive only rehabilitation (n = 11) or rehabilitation combined with VNS (n = 9). The VNS device was implanted on the left vagus nerve and connected to a pulse generator implanted at the level of the chest. All patients received 2-h rehabilitation sessions, three times a week, for 6 weeks. The VNS group received stimulation that was manually triggered wirelessly by the therapist during each repetition of the rehabilitative task (reach and grasp, gross movement, simulated eating, handle turning, etc.). Twenty-two adverse events (AEs) were observed including six patients with hoarseness. Despite the small sample size, per-protocol analysis revealed a significant improvement in performance on the Fugl–Meyer Assessment—Upper Extremity in the VNS group when compared to the rehabilitation alone group. However, no differences were seen on intent-to-treat analysis or on any of the secondary endpoints, which were more functional in nature. Further clinical and preclinical studies will help elucidate the potential role of VNS for the treatment of poststroke motor impairment.
Epidural Cortical Stimulation
Epidural cortical stimulation (ECS) was initially investigated as a treatment for central pain syndromes. In these studies, ECS not only demonstrated efficacy in reducing chronic pain but improvements in motor function as well ( ). Although ECS has not been widely adopted for the treatment of chronic pain ( ), interest in its purported motor benefits prompted preclinical investigations for poststroke hemiparesis. Early studies were promising, with application in both rodent and nonhuman primate models of stroke demonstrating that significant improvements in motor performance could be achieved ( ). In one of the first preclinical studies, Adkins-Muir et al. investigated the effects of 50 or 250 Hz ECS paired with rehabilitation in a rodent model of IS induced by ET-1 injection into the sensorimotor cortex ( ). The investigators found that 50 Hz stimulation significantly improved the rate of recovery of the paretic limb that was paralleled by an increase in the dendritic expression of microtubule-associated protein-2 in the perilesional cortex, which served as a marker of neuronal structural plasticity. Extending these findings, Kleim et al. showed that stimulation paired with training on the single pellet reaching task, a common tool to measure rodent forelimb function, resulted in significant expansion of motor representation of the affected limb in the perilesional cortex ( ). A comprehensive study, employing both cortical stimulation and evoked potentials recorded via bipolar electrodes, provided further support for the efficacy of cortical stimulation combined with rehabilitation in rats ( ). Using a primate model, Plautz et al. developed cortical maps prior to and several weeks following electrocautery lesion of the primary motor cortex subserving hand function. Compared to sham-treated animals, stimulation-treated animals demonstrated improved motor performance and increased hand representation in the areas underlying the stimulation electrodes ( ).
Following the positive results of the earlier-mentioned preclinical studies, clinical trials studying ECS as motor cortex stimulation (MCS) in chronic IS commenced. In the first trial, in which 10 patients who were a minimum of 4 months poststroke were enrolled, the MCS group (n = 6) demonstrated statistically significant improvements on the Fugl–Meyer Assessment and the Stroke Impact Scale relative to the untreated group ( ). Although there were two perioperative complications (one infection, one lead breakage), no complications attributable to stimulation were observed (e.g., neurologic deficits or seizures). The subsequent, Phase II trial enrolled 24 patients (12 treatment, 12 control) ( ). In this study, one seizure was reported in the treatment group following implantation, but prior to the initiation of MCS. Once again, the group receiving MCS and rehabilitation demonstrated significant improvements on the Fugl–Meyer Assessment as well as the Box-and-Block test, relative to the rehabilitation only group at 6 month follow-up ( ). In the pivotal Phase III trial, 146 patients were enrolled (91 treatment, 55 controls). Despite the promising results of the prior trials however, this study found no difference between the group receiving stimulation and rehabilitation and the group receiving only rehabilitation ( Fig. 95.2 ) ( ). Following the failure of this trial, several publications have attempted to elucidate the factors leading to this outcome ( ). Potential contributions to the lack of efficacy include: (1) Inappropriate target selection or target localization; (2) A lack of sufficient, surviving perilesional cortex in patients with diffuse ischemic lesions spanning multiple functional regions; (3) Lack of viable descending motor pathways. In the Phase I and II trials, which showed promising functional improvements, 100% and 42% of patients, respectively, demonstrated evoked movements with TMS or ECS pretreatment, compared to only 16% of the patients enrolled in the Phase III trial. In support of this hypothesis, retrospective analysis of the patients enrolled in the trial revealed that those who responded to therapy were more likely to have a smaller proportion of the corticospinal tract injured by stroke and have preserved motor evoked responses to MCS ( ); (4) Focal stimulation activating a relatively small volume of cortex. The volume of the human precentral gyrus is approximately 100-fold larger than that of animals in preclinical studies. The stimulation contact area of the device used in the Phase III trial, however, was only four to seven times larger than the arrays typically used in animal models, which may have prevented adequate modulation of spared perilesional cortex ( ); and (5) The complex, gyral, and sulcal anatomy of the human cortex, with neurons organized in multiple orientations relative to the epidural electrodes. Because the response of a given neuron to stimulation is dependent upon its orientation relative to source of electrical stimulation, it is possible that ECS produced a net neutral or negative due to the variable orientation of neurons in relation to the epidural array ( ).

Subsequent, preclinical trials investigating ECS/MCS have attempted to provide evidence to support the hypothesized reasons that led to the negative outcome. Boychuk et al. investigated the response to ECS in two different stroke models, one in which ET-1 was injected in the vicinity of the MCA in order to produce a cortical and lateral striatal infarct and another in which it was injected into a subcortical region causing infarction of the posterior thalamus and internal capsule. The authors found that while stimulation was effective in promoting recovery in the MCA infarct model, no such benefits were seen in the subcortical injection model, thus highlighting the importance of infarct extent and location, likely with respect to preservation of descending motor pathways, in determining response to therapy ( ). In revisiting cortical stimulation in a primate model of focal ischemic lesion in the distal forelimb representation of M1, Plautz et al. found that stimulation initiated 7 weeks after lesion did not provide any benefits in terms of skilled reaching performance. Furthermore, while the control group receiving rehabilitation alone demonstrated expansion of distal forelimb cortical representation, the stimulation group did not, providing, in the authors’ words, “cautionary implications for translation of cortical stimulation/rehabilitation therapy to clinical populations” ( ). To address concerns regarding the optimal targeting of cortical stimulation to produce benefits, Boychuk, Adkins, and Kleim compared the effectiveness of distributed versus focal cortical stimulation. Stimulation targeted directly above M1 showed no benefit over rehabilitation alone while distributed stimulation did improve motor outcomes ( ). The studies support several of the hypotheses regarding the role of infarct location, the timing of stimulation, preservation of descending, motor tracts, and the necessity of stimulating wide areas of perilesional cortex in order to produce a beneficial improvement in motor performance.
Deep Cerebellar Stimulation
Deep brain stimulation (DBS) of the cerebellar dentate nucleus is an alternative to NIBS and ECS that addresses several of the limitations encountered by those therapies. The subsequent section will detail how stimulating a relatively compact node in a strongly interconnected network of projections between the cerebral cortex and the cerebellum obviates many of the shortcomings of ECS by providing an easy to localize target that projects to and activates broad regions of cerebral cortex, regardless of the spatial orientation of the target neurons; thereby, increasing the probability that stimulation will activate spared, perilesional cortex crucial for functional reorganization and recovery. The theoretical basis for this approach is rooted in reversing diaschisis, promoting perilesional excitability and perilesional plasticity after injury ( ).
Diaschisis refers to a reduction in metabolism, blood flow, and activity of a region distant from, yet functionally connected to a focal brain lesion. Crossed cerebellar diaschisis (CCD) in particular refers to a decrease in activity of the cerebellar hemisphere contralateral to a cerebral lesion secondary to the interruption of reciprocal projections between the contralateral cerebral hemisphere and the cerebellum ( ). As a result of deafferentation following cortical injury, cerebellar activity decreases causing a subsequent decrease in excitatory output from the cerebellum back to the cortex. Chronically, CCD may result in significant atrophy of the affected cerebellar hemisphere. Studies using nuclear imaging have reported frequencies of CCD ranging from 16% to greater than 50% ( ) and the presence of CCD is associated with worse functional outcomes and decreased cortical excitability ( ). Given the strong, reciprocal connections between the cortex and cerebellum and the parallel changes in metabolism and activity in a large portion of patients following stroke, reactivation of this pathway presents an exciting target for therapeutic intervention.
The dentate nucleus is the largest of the deep, cerebellar nuclei. It receives input from the lateral, cerebellar hemisphere and afferent projections from the cerebrum via the corticopontocerebellar pathway (CPC). The primary output of the dentate is the dentatothalamocortical pathway, projecting from the cerebellum to the contralateral, ventral lateral pars caudalis (VLc), ventral posterior lateral pars oralis (VPLo), and medial, dorsal (MD) thalamic nuclei before terminating in a variety of cortical regions ( ). Importantly, via its widespread thalamic targets, the dentate projects not only to the motor cortex, but also to premotor and parietal cortices ( ). The work of Di Lazzaro, Werhahn, and Rispal-Padel, using single- and short-pulse stimulation of the dentate has provided direct evidence that cerebellar stimulation is capable of eliciting a cortical response ( ). Electrical stimulation of the dentate thus holds the potential to activate this natural fiber pathway and its downstream targets. As the dentate projects to a large area of the cortex, including M1, premotor, frontal, and parietal areas, dentate stimulation has the potential to activate a significantly larger area of cortex than NIBS or ECS.
Building off this anatomic and pathophysiologic foundation, DBS of the dentate nucleus, referred to as the lateral cerebellar nucleus (LCN) in the rodent, was investigated for its benefit in promoting poststroke, motor function ( ). In the first study investigating the effects of LCN DBS, rats were trained on the Montoya staircase task prior to undergoing a three vessel-occlusion-model stroke and subsequent implantation of a bipolar electrode in the contralesional LCN. Animals were randomized to receive 6 weeks of sham, 10, 20, or 50 Hz stimulation, initiated 2 weeks following ischemia. We found that 20 Hz stimulation produced a statistically significant improvement in performance on the Montoya task relative to sham ( ). Guided by the hypothesis that changes in cortical excitability underlie the behavioral improvements derived from LCN DBS and the frequency dependent effect on recovery, subsequent investigations in naïve rats combined LCN DBS with intracortical microstimulation to elicit MEPs. In this study, hindlimb MEPs were evoked via intracortical microstimulation while the subject received sham, 20, 30, 40, 50, or 100 Hz stimulation in the contralateral LCN. Consistent with findings from preclinical ECS studies, high frequency (100 Hz) stimulation reduced cortical excitability while 30 Hz cerebellar stimulation provided maximal facilitation of cortical excitability ( Fig. 95.3 ) ( ). In poststroke animals, although MEPs were reduced overall relative to naïve animals, LCN DBS was still capable of enhancing cortical excitability ( ). We then replicated and extended prior findings in the focal ET-1 model of ischemia, where 30 Hz LCN DBS was found to improve performance on the pasta matrix task ( ). Therapeutic efficacy was further associated with increased expression of synaptophysin in the perilesional cortex when compared to sham controls. Strengthening the hypothesis that changes in cortical plasticity underlie functional recovery, a follow-up study by Cooperrider et al. demonstrated that poststroke LCN DBS resulted in increased distal forepaw representation. In addition, Western blot analysis showed increased expression of markers of LTP and plasticity, namely synaptophysin, NMDAR1, CaMKII, and PSD95, while 3D electron microscopy revealed a twofold increase in synaptic density in animals receiving stimulation ( ).
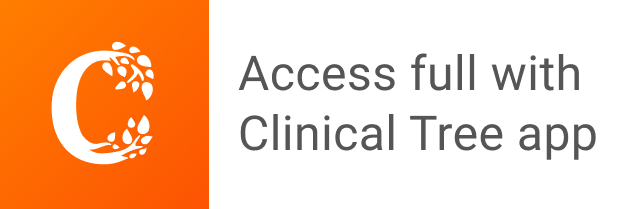