Introduction
Brain functional and structural integrity depends on a constant supply of oxygen and glucose through cerebral blood flow (CBF) to the cerebral microcirculation. Accordingly, adequate function of the cerebral microcirculation is a prerequisite for sufficient oxygen (O 2 ) delivery to brain cells. Under normal conditions, the brain makes adjustments in local CBF to support metabolic demands and prevent energy failure. However, if an imbalance occurs between supply (CBF) and consumption (cerebral metabolic rate of oxygen [CMRO 2 ]), cerebral ischemia may develop. If CBF falls below a critical level and oxygen extraction has reached its limit, electrical and certain metabolic functions are lost, and irreversible brain damage (infarction) may occur. The development of tissue infarction is related to both the degree and duration of CBF reduction. This important concept implicates that certain thresholds exist below which infarction occurs and that there is a limited period of time that ischemia may still be reversible (“therapeutic window”). Therefore, it may be helpful to have monitoring instruments that can detect cerebral ischemia before these thresholds are crossed and allow the clinician to take measures in a timely manner to prevent the development of brain infarction. In addition instruments are needed that provide information on morphology and function of the cerebral microcirculation to better understand the pathophysiology of cerebral ischemia. In particular, continuous assessment of CBF helps to assess cerebral autoregulation and pressure-reactivity. This is important because both can be independent factors associated with outcome after acute brain injury.
Current treatment modalities to prevent or reduce ischemic brain damage are mostly guided by parameters such as intracranial pressure (ICP), cerebral perfusion pressure (CPP), brain tissue oxygen tension (P bt O 2 ), and cerebral metabolism and not by direct CBF measurements. Direct monitoring of CBF could provide the opportunity to diagnose and to correct insufficient CBF before deficits in tissue oxygenation and metabolism are recognized. Local microvascular CBF can be measured continuously and invasively in the neurocritical care unit (NCCU) with laser Doppler flowmetry (LDF) and thermal diffusion flowmetry (TDF). Many years of clinical experience have been obtained with these monitoring modalities. This chapter gives an overview of LDF and TDF: history, technology, validation, clinical applications, and developments are described. In addition, orthogonal polarizing spectral (OPS) imaging and its successor sidestream dark field (SDF) imaging are discussed as examples of optical techniques that allow direct observation of the human microcirculatory morphology and function.
Laser Doppler Flowmetry
Bedside evaluation of CBF has been a long-standing goal in neurocritical care. Potentially, it could assess flow alterations, measure the effect of therapy, and evaluate the integrity of cerebral autoregulation. LDF was first introduced in 1977 to measure cutaneous blood flow, and after commercialization in the early 1980s, a stable and steadily growing clinical application has occurred. LDF provides continuous, qualitative measurements of microvascular perfusion intraoperatively or in the intensive care unit as a bedside monitoring device; this is attractive to clinicians working with critical neurologic diseases in which cerebral ischemia is frequently encountered. However, the routine clinical applicability of this optical technique remains logistically complex and is hindered by various artifacts and is limited by the absence of quantitative regional cerebral blow flow (rCBF) values. Consequently diagnostic and prognostic values have not been reported and instead LDF is best used as a trend monitor in individual patients.
In LDF, a fiber-optic laser probe with a diameter of 0.5 to 1 mm is applied to the surface of the brain or within the brain to illuminate a tissue volume of approximately 1 mm 3 with monochromatic laser light of various wavelengths from 670 to 810 nm. As light impinges on the brain tissue, photons are scattered and Doppler shifted in a random fashion via dynamic red blood cells and stationary tissue cells. A fraction of scattered shifted and nonshifted photons is detected by photoreceptors, which generates an electric signal. This electric signal contains information about power and frequency proportional to the volume or concentration (fraction of total photons that is frequency shifted) and velocity of red blood cells (signal frequency broadening) ( Fig. 31.1 ). The commercially available laser Doppler signal processors use Bonner and Nossal’s algorithm to analyze the signal and to produce a flow output. The product of volume and velocity scales linearly with the perfusion (CBF) and is expressed in arbitrary units (AUs).

The principles of LDF measurements are implemented in surface (cortical) probes and intraparenchymal (subcortical) probes to obtain real-time continuous local microvascular arbitrary flow values from capillaries at the cortical surface and within the brain. The cortical probe consists of a thin contoured disk with a long optical fiber that is connected to a monitor. The probe is positioned subdurally and so can be prone to motion artifacts caused by brain pulsations or subsidence of brain swelling. To avoid disturbances of the flow values, the probe should be placed in a relatively normal brain region without large vessels. The intraparenchymal probe is introduced through a fixed bolt in the skull, which eliminates motion artifacts, and advanced to the white matter. The benefit is that measurements are obtained from the same local brain region as other intracranial monitoring devices such as ICP and microdialysis, which may improve interpretations of those parameters. The main manufacturers of laser Doppler instruments are Perimed AB (Stockholm, Sweden), Moor Instruments Ltd. (Axminster, UK), Vasamedics Inc. (St. Paul, MN), Transonic Systems Inc. (Ithaca, NY), Oxford Optronix Ltd (Oxford, UK) and LEA Medizintechnik (Giessen, Germany).
LDF provides a reliable measurement of microvascular (local) rCBF as demonstrated by multiple validation studies. A high correlation is found between LDF and several other blood flow monitoring techniques such as xenon (Xe) clearance method, radioactive microspheres, hydrogen clearance technique, iodoantipyrine method, and thermal diffusion. Furthermore, it has been successfully applied in the brain-injured patient to assess autoregulation, carbon dioxide (CO 2 ) reactivity, and response to therapeutic interventions and to detect ischemic insults. For example, Schubert et al. have used LDF studies to detect hypoperfusion independent from ICP in the early and the acute stages of subarachnoid hemorrhage (SAH).
In the intensive care unit LDF can be used to help predict outcome. For example, Lam et al. continually assessed autoregulation with LDF in 31 comatose head-injured patients. A linear relationship between cortical LDF and CPP was used as an indicator for loss of autoregulation. Intact autoregulation correlated with a good outcome and persistent loss of autoregulation with a poor outcome. However, loss of contact with the cerebral surface was responsible for unreliable data in 16% of patients. Smielewski et al. assessed the transient hyperemic response after traumatic brain injury using LDF and found that patients with an unfavorable outcome had significantly lower LDF readings than patients with a favorable outcome. The concomitant use of LDF with transcranial Doppler (TCD) to help detect delayed cerebral ischemia confirmed that high-flow velocities and ischemia are not often related. LDF also can be used intraoperatively to assess microvascular flow alterations in a region of interest during aneurysm surgery, arteriovenous malformation (AVM) resections, and bypass procedures and to interpret the physiologic changes associated with the surgery, understand the cortical microcirculation, and help predict postoperative hyper- or hypoperfusion syndromes. In addition LDF studies have been used to assess blood flow in pericranial flaps during reconstructive surgery.
Despite LDF’s excellent temporal and dynamic resolution with ultra short time responses to fluctuations in CBF there are several limiting factors that may compromise data quality. Different inter- and intraindividual LDF signals can be generated by device-related factors such as calibration procedure, monochromatic wavelength, and probe configuration. The penetration depth of LDF is approximately 1 mm, depending on wavelength distance between transmitting and receiving fibers (fiber separation). A shorter wavelength or a smaller fiber separation will give lesser tissue penetration. Artifacts can be induced by a large number of external derangements such as the heterogeneity of microvascular architecture, changes in hematocrit, tissue or probe motion, room temperature, strong external light, and sound. LDF only measures a very small area of brain tissue that may not necessarily represent the global CBF due to the heterogeneity of the cerebral microcirculation. Multichannel instruments and laser Doppler perfusion imaging were introduced to overcome potential limitations associated with temporal and spatial resolution. These factors make inter- and intraindividual comparison of data difficult. Therefore a detailed standardized measurement protocol is a prerequisite to obtain reproducible and comparable LDF data. This protocol includes at least device-related technical data, anatomic site selection, and frequent calibrations (every 15 minutes) to circumvent improper data collection due to changes in hematocrit, motion, room temperature, strong external light, and sound. Finally, the inability of LDF to produce absolute CBF values hinders the definition of a threshold value that indicates cerebral ischemia; that is to say, LDF is a trend monitor.
Thermal Diffusion Flowmetry
TDF is based on thermal conductivity of brain tissue to measure quantitative blood flow. In 1933, Gibbs demonstrated that changes in CBF could be detected with a heated thermocouple and Carter and Atkinson developed a mathematical model to calculate absolute rCBF values. In 1973 TDF was introduced and validation studies showed that rCBF values obtained by TDF are in good agreement with rCBF values obtained in xenon-enhanced computed tomography (Xe-CT) studies and the hydrogen clearance method.
Currently two technical systems are commercially available that use the temperature difference measured between a thermistor and temperature sensor to calculate CBF. A small Silastic probe(s) (Flowtronics, Inc., Phoenix, AZ) with two gold disks can be placed subdurally on the cortex to measure superficial rCBF. During placement large vessels and pathologic tissue need to be avoided to prevent artifacts. A confidence factor can be calculated by turning off the heater to determine proper contact with the cerebral cortex. A confidence factor of 0.8 or higher is considered to represent reliable data. When the correct position is confirmed the dura can be closed. Recently, an intraparenchymal thermal diffusion microprobe (Hemedex Inc., Cambridge, MA) has been introduced and validated that eliminates artifacts caused by loss of surface contact and obtains rCBF measurements from the same vascular territory as other intraparenchymal monitoring devices (i.e., ICP, P bt O 2 ). This novel TDF microprobe consists of a thermistor in the tip of the probe and a temperature sensor located a few millimeters proximal that provides sensitive and continuous absolute rCBF values even in the case of minor flow changes ( Fig. 31.2, A ). It is inserted through a bolt fixed in the skull with the tip approximately 25 mm below the dura in normal brain tissue, which can be verified with a CT scan. Automatic recalibration occurs approximately every 30 minutes; this results in a loss of data for 2 to 5 minutes. In addition, significant baseline shifts of CBF that occur after each recalibration independent of hemodynamic stability are a concern. Both systems have a built-in safety mechanism that prevents CBF measurements when temperature is greater than 39 o C. This safety mechanism thus interrupts monitoring of the cerebral perfusion during periods of high fever that are frequent in patients with severe closed head injury or subarachnoid hemorrhage.

TDF provides in real time the dynamic changes in actual cerebral perfusion, that is, quantitative data. Therefore this technique can be applied intraoperatively and at the bedside to prevent, detect, or treat abnormalities in cerebral perfusion and so alter clinical outcome. Superficial (cortical) rCBF values between 40 to 70 mL/100 g/min are normal and values less than 20 mL/100 g/min or greater than 70 mL/100 g/min represent respectively ischemia and hyperemia. The TDF microprobe measures subcortical white matter perfusion, and a mean TDF value of 18 to 25 mL/100 g/min is considered normal.
In critically ill brain-injured patients, rCBF monitoring with Silastic TDF sensors can help predict outcome. Specifically, patients with a significant increase in rCBF from baseline values, have a good outcome, whereas patients with initial very low values and those without an increase in flow from baseline have a poor outcome. In this study, however, reliable data could only be obtained in 37 of 56 patients because the routine application of this technique is not always easy. rCBF can complement parameters such as CCP, ICP and P bt O 2 , and so facilitate the interpretation of these parameters. In closed head injury, monitoring of CPP and treatment of low CPP is considered necessary to prevent cerebral ischemia. However, with a disrupted autoregulation, a CPP greater than 70 mm Hg may still be associated with ischemic events, and therefore restoration and maintenance of adequate perfusion could be performed under guidance of continuous rCBF monitoring. Likewise use of a Hemedex probe can help evaluate cerebral autoregulation and CO 2 vasoreactivity by allowing for calculation of local cerebral vascular resistance (CPP/rCBF) in response to a blood pressure or hyperventilation challenge. Alternatively continuous monitoring of TDF-CBF, CPP, and brain oxygen allows calculation of flow- and oxygen-related autoregulation indices. TDF provides absolute values (unlike LDF) and therefore has the ability to distinguish between elevations of ICP associated with ischemia or hyperemia. A study of SAH and traumatic brain injury patients found a correlation between CBF measured by the TDF microprobe and P bt O 2 , indicating that tissue oxygen levels were determined in part by regional CBF. This same study showed that loss of data due to recalibrations or periods of fever is a concern. The TDF microprobe allowed CBF measurement 64% of the time when P bt O 2 monitoring was possible.
In SAH, TDF can be used to help detect vasospasm and monitor for delayed cerebral ischemia. Vajkoczy et al. found a threshold of 15 mL/100 g/min to diagnose symptomatic vasospasm had a sensitivity of 90% and specificity of 75%. In addition, the effectiveness of treatment can be determined with continuous rCBF monitoring. Therefore TDF represents a useful and effective technique to help detect delayed cerebral ischemia in SAH. Whether the use of this technique in the NCCU can lead to improved clinical outcome will need further study.
TDF can be used intraoperatively to study hemodynamic changes that occur in the surrounding normal brain tissue during aneurysm clipping, bypass surgery ( Fig. 31.2, B ), or resections of AVM’s and brain tumors. Acute changes in cortical CBF with vascular manipulations during aneurysm clipping have been demonstrated with TDF. Furthermore, increased perinidal cortical CBF values were found with TDF and LDF after AVM resection. Detection of hyperemic values may indicate pending normal perfusion pressure breakthrough, and therapeutic interventions (i.e., barbiturate coma) can be instituted promptly to avoid further brain tissue damage. A decrease in peritumoral CBF was found before tumor removal, which recovered after completion of the resection, indicating that compression of the peritumoral microvasculature was responsible for a decrease in blood flow. During epilepsy surgery, it was demonstrated that normalization of peri-ictal bihemispheric cortical CBF occurs in temporal lobe epilepsy from a reduction of cortical CBF interictally.
Limitations associated with TDF include “nonmonitroing” during recalibration and fever, sensor displacement or loss of brain surface contact, and that only a small area of brain tissue is monitored (20 to 30 mm 3 ). Therefore global ischemia or focal ischemia in other unmeasured flow regions will be missed. This limitation may be overcome partially by the use of multiple sensors on a strip or placement of multiple intraparenchymal microprobes. Complications associated with TDF microprobes are very rare and similar to other implanted intraparenchymal probes (i.e., an approximate 1% risk of significant bleeding or infection).
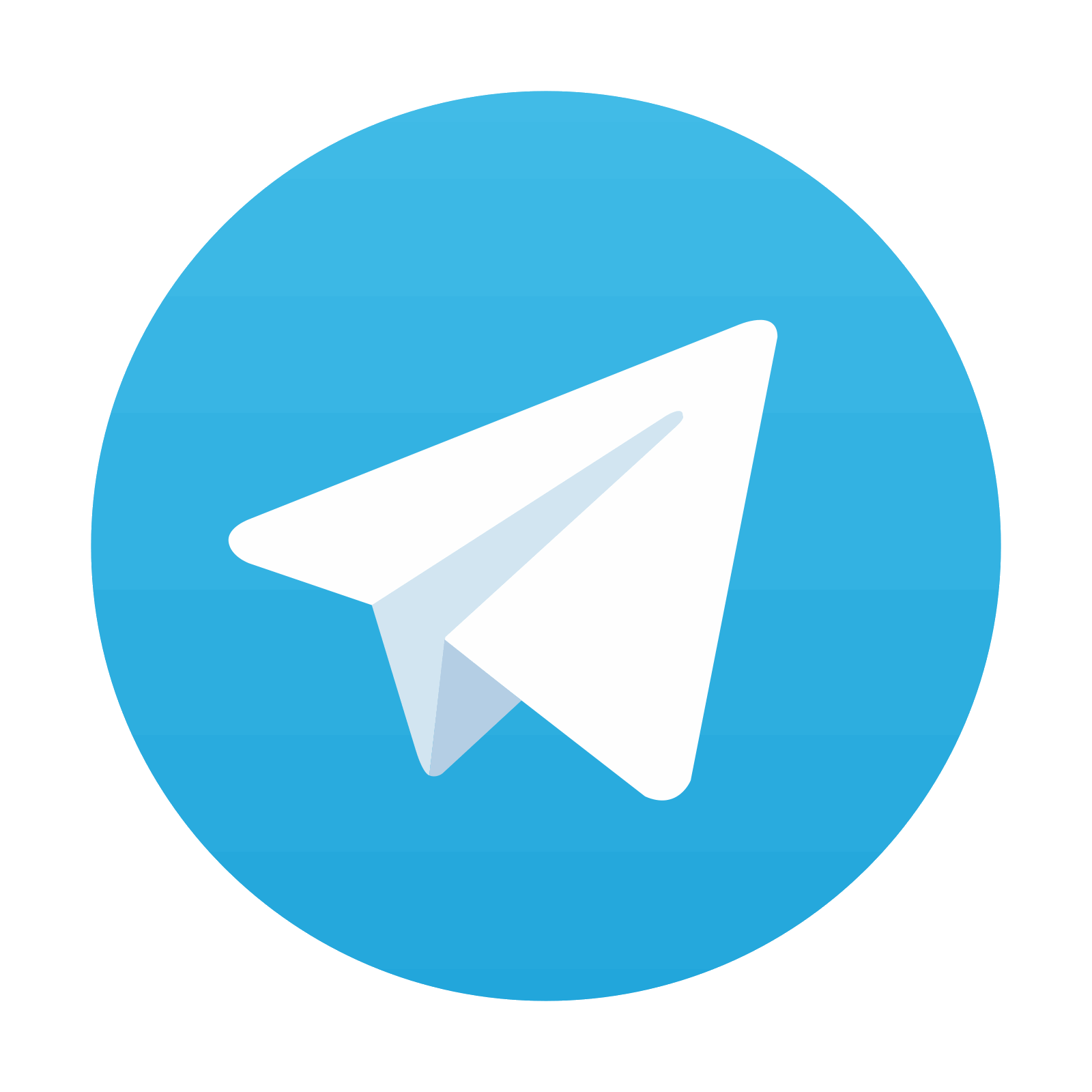
Stay updated, free articles. Join our Telegram channel
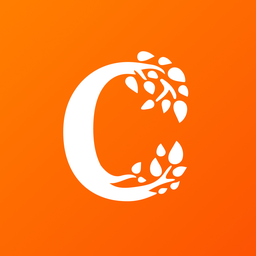
Full access? Get Clinical Tree
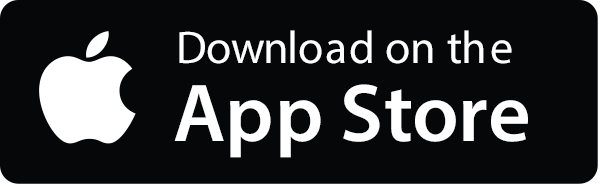
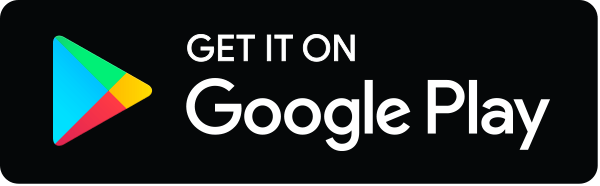