Introduction
The link between Down syndrome (DS), aging, and Alzheimer’s disease (AD) is well established (see Chapter 1). In people with DS, dementia prevalence increases with age. In those who are age 60 and older, it is estimated to be as high as 77% [1]. In addition to accumulation of the two hallmark pathologies of AD—amyloid plaques and neurofibrillary tangles—a host of AD-related changes take place at all levels of the nervous system (see Chapter 2). These include neuron loss, white matter pathology, cerebrovascular pathology, oxidative damage, and neuroinflammation [2]. A number of these pathologies can be examined in vivo, and magnetic resonance imaging (MRI) technology can offer suitable noninvasive tools to derive disclosing biomarkers that may precede and predict clinical symptoms.
A major challenge for biomarker development is determining whether the brain changes observed in older people with DS are specific to AD. There are several developmental confounds researchers must consider when comparing people with DS to neurotypical people with sporadic AD [3]. There are developmental differences in brain structure, function, and metabolism, in addition to cognitive impairments secondary to intellectual disability. These factors may confound the detection of AD-related cognitive decline in those with DS. For example, verbal memory deficits are often found in DS but are also frequently used as an outcome measure indicating early cognitive impairment in sporadic AD. The rate and order of clinical symptom presentation may differ between those with DS and those with sporadic AD. For example, the onset of dementia occurs at a relatively younger age for people with DS [4] (see Chapter 15). DS represents an at-risk model for AD with early vulnerability to neurodegeneration, similar to autosomal dominant familial AD, which inevitably leads to dementia [5].
Neuroimaging is an important tool for understanding the aging brain and neurocognitive decline. It also enables identification and validation of AD biomarkers. Using neuroimaging to study people with DS plays a critical role in early diagnosis and thorough disease monitoring in this population. In turn, studying AD in people with DS can improve diagnosis of AD in the general population. Identifying the neural changes that best predict dementia in DS may help clinicians diagnose at-risk people earlier in the disease course and will add new targets for therapeutic intervention [3]. Multimodal neuroimaging allows us to map the trajectories of AD-related brain changes, each of which may have a different biological basis. But classic biomarker models, such as those proposed by Jack et al. [6], do not necessarily account for the distinct trajectories detected by different neuroimaging modalities. Other models have begun to account for these differences, but more research is needed to fully characterize the spatiotemporal progression of disease biomarkers [7, 8]. See Fig. 1 for an example of a multimodal MRI AD biomarker model. In particular, emphasis should be placed on longitudinal studies, which can track changes over time beginning before an individual is symptomatic.

In this chapter, we review several neuroimaging modalities currently being used to study aging and AD in people with DS and discuss their strengths and limitations. We discuss the existing literature on AD in DS and highlight future directions for research.
Structural neuroimaging
Technical background
Structural magnetic resonance imaging (MRI) is widely used for research and clinical purposes across various cohorts and patients, providing excellent anatomical detail and a strong gray/white matter contrast. It typically utilizes short scan times, allowing for quick image acquisition with potential diagnostic utility. While structural imaging of any part of the body is possible, brain imaging is well suited for quantitative and qualitative assessment of neuropathologies, because processes that are believed to be pathological in nature are often described in terms of anatomical location, cortical thickness, regional volumes, vascular lesions, and morphological alterations. Therefore, structural imaging has many potential applications for people with DS who are profoundly vulnerable to AD-related neuropathology and dementia.
MRI can generate high-resolution (0.5–1 mm) images of water-containing tissue (made up of hydrogen atoms, i.e., protons). Application of a strong magnetic field (typically 1.5–3 T for humans and up to 21 T for small animals) and pulses of radiofrequency waves can manipulate the electromagnetic fields and energy state of protons in the body, which emit energy that can be converted to signals and subsequently converted to images. A wide array of processing and analytical techniques has been developed for different types of tissues. Different MR pulse sequences generate images with varying signal contrast and intensities. T1-weighted sequences involve a short repetition time (TR, < 1000 ms) and echo time (TE, < 30 ms). T2-weighted sequences involve a long TR (> 2000 ms) and TE (> 80 ms). Fluid-attenuated inversion recovery (FLAIR) sequences are based on T2-weighted with hypointense (i.e., suppressed) cerebrospinal fluid (CSF) signal. Gradient recalled echo (GRE)-T2* (T2 star)-weighted images involve generally shorter TR and TE than spin-echo sequences (T1 or T2), allowing rapid imaging acquisitions.
On T1-weighted images (Fig. 2A and D), water (e.g., CSF, edema, infarction, infection, inflammation) appears dark, while fat (e.g., lipids in the myelin-sheathed white matter) appears bright. T1 images are optimized for distinguishing gray matter (appears as dark gray on the image) from white matter (appears as lighter gray on the image), revealing anatomical details with high fidelity. For example, T1-weighted scans are often used to measure and assess changes in volumes or cortical thickness of various regions known to undergo structural neurodegeneration.

On T2-weighted images (Fig. 2B), fat appears dark and CSF appears bright, providing an optimized measure of water content. Because disease-related pathologies are often associated with changes in water content, T2-weighted images are useful for illustrating pathological signatures. For example, FLAIR images (Fig. 2C) are particularly useful for delineation of cerebral or vascular lesions such as white matter hyperintensities (WMHs) which typically manifest near ventricles (periventricular caps) and are quite common with aging and neurological disease. T2*GRE imaging or susceptibility weighted imaging (SWI) is a form of T2-weighted image that is susceptible to calcium or iron. On T2* images, bone (rich in calcium) and blood (rich in iron) appear dark, making it useful for quantification of major classes of small and large vessel cerebrovascular abnormalities, including infarcts, microbleeds, or other vascular pathologies.
In the absence of obvious visible pathological changes, it is more difficult to link volumetric or shape changes to particular disease states. Thus, structural MRI by itself is insufficient for a diagnosis of neurodegenerative disease. It is also challenging to determine the neural source of volume or thickness loss (e.g., cell loss vs. dendritic and synaptic loss) without ultrahigh-resolution scanning at 7 T or higher, which is not feasible for most institutions. Of all MRI scan types, however, structural MRI is the easiest to harmonize across sites and scanners, making it the neuroimaging biomarker of choice for large multisite trials and observational studies.
Specific challenges in the use of structural MRI in people with DS include the high degree of head movement, which leads to motion-related artifacts that reduce the effective scan resolutions and induce blurriness and other uncertainties that compromise analyses. Additionally, anatomical brain structure in people with DS undergoes developmental changes that may require the use of specialized anatomical atlases and analytic tools. Applying standard atlases based on normative brains may result in poor fit and exaggeration of pathological findings.
Structural brain abnormalities in aging brain of DS
Converging evidence from structural neuroimaging studies suggests altered brain morphology and volume with increasing age in the general population. The medial temporal lobe, for example, is considered an exceptionally vulnerable region that is susceptible to age- and AD-related pathology. MRI studies have shown that hippocampal and entorhinal volume decline with increasing age and predict age-related performance deficits on memory tasks [7, 9–11]. It appears that these changes are not the result of cell loss but likely are driven by synaptic and dendritic dysfunction. Hippocampal volume loss has been noted in the early stages of AD and has been linked to cognitive impairment [12–14].
Virtually all people with DS over the age of 40 have neuropathological hallmarks (neuritic plaques and neurofibrillary tangles) sufficient for a diagnosis of AD in general population [1]. However, identification of age- and AD-related brain changes in people with DS may be challenging because of preexisting developmental differences in brain structure. The relationship between structural measures and cognition is also unclear and difficult to measure due to the existing intellectual disability, which complicates standardized neuropsychological assessment. Nevertheless, structural MRI studies in older people with DS have shown a pattern of pathological changes that is generally (1) greater than the pattern observed in older neurotypical individuals and (2) similar to the pattern of tissue loss in the early stages of AD in older neurotypical individuals. We describe these results in further detail later.
Morphological and volumetric abnormalities
Developmentally, DS is often characterized by smaller brain weight, diminished regional volumes, and a simplified superior temporal gyrus compared to neurotypical individuals [15–19]. Consistently, older people with DS are found to have smaller intracranial volumes and larger ventricles than neurotypical people of similar age [20, 21]. Early MRI studies examining people with DS (ages 22–51) used manual tracing methods and found reduced brain regional volumes and accelerated brain aging when compared to neurotypical controls. Weis et al. reported smaller volumes in the whole brain, cerebral cortex, white matter, and cerebellum using the stereologic method of Cavalieri [22]. Kesslak et al. reported smaller areas of frontal cortex, cerebellum, and the hippocampus (but a larger area of parahippocampal gyrus [PHG]) in people with DS compared to healthy controls [20]. Other work has shown that people with DS have smaller cerebral and cerebellar hemispheres, ventral pons, mammillary bodies, hippocampus, and several cortices (dorsolateral prefrontal, anterior cingulate, inferior temporal, parietal, pericalcarine) than neurotypical controls [23].
More recent MRI studies examined elderly people with DS across a wider age range (up to 72 years of age) and used operator-independent volumetric analyses (voxel-based morphometry) to describe cross-sectional differences between nondemented people with DS and neurotypical controls. One study showed that in a nondemented group with DS, smaller volumes of the hippocampus, amygdala, and posterior PHG were associated with advanced age, an effect that was not noted in healthy controls [24]. Another study showed reduced gray matter volume in cerebellum, left medial frontal lobe, right superior and middle temporal lobe, and anterior and middle cingulate gyri as well as increased white matter volume of the PHG in those with DS [25]. Haier et al. showed that in a small sample of nondemented, middle-aged people with DS, decreased gray matter volume and higher glucose metabolic rates in the temporal and frontal cortices as well as subcortical regions were associated with cognitive impairment [26].
To examine brain structural abnormalities related to dementia in DS, MRI studies compared demented and nondemented people with DS. Pearlson et al. showed that demented people with DS have more generalized atrophy, medial temporal lobe shrinkage, reduced volumes of the left hippocampus and left amygdala, and increased ventricular volumes than nondemented people with DS [27]. Aylward et al. also noted volume reduction in the hippocampus and amygdala in demented people with DS [28]. Sabbagh et al. also reported decreased volumes in the hippocampus, basal ganglia, medial temporal lobe, prefrontal cortex, lateral parietal cortex, and precuneus in people with DS diagnosed with AD [29]. Although the sample size of demented people with DS was small in most of the studies, the results suggest that aging people with DS exhibit a wide range of severity in cognitive impairment and MRI-based brain abnormalities.
Volumetric measures have been linked to measures of memory function before onset of dementia, suggesting that neuroimaging measures may be predictive of subsequent memory deficits in DS (as in normal aging). For example, Krasuski et al. showed that amygdala and hippocampal volumes were positively associated with memory performance, as measured by Down Syndrome Mental Status Examination (DS MMSE) and the Hidden Object Memory Test, but not with nonmemory performance [24]. Likewise, Teipel et al. found a positive association between gray matter in the precuneus, left lateral temporal cortex, left hippocampus, and right prefrontal cortex and verbal memory performance (as measured by DS MMSE) after accounting for age [30].
Generally, MRI studies have demonstrated that aging people with DS have more extensive neurodegeneration than neurotypical individuals. The most prominent reductions are noted in the medial temporal lobes, including the hippocampus, which resembles the spatiotemporal pattern of neurodegeneration in sporadic AD. A recent study with a large sample of people with DS (n = 69) showed that hippocampal volume was largest in nondemented people and decreased with diagnosis severity, after correcting for age and intracranial volume differences [31]. Interestingly, some earlier studies noted larger PHG volume, which may indicate altered neural circuits in people with DS that may subsequently affect cognition and function [20, 23, 25] and may be attributable to developmental differences in neurogenesis in DS rather than advancing age [32].
The specific pattern of age-related differences varied across studies. Some studies found age-related reductions in regional brain volumes in frontal, temporal, and parietal lobes [20, 24, 30, 33], while other studies found that hippocampal volume change was independent of age [23, 25, 30]. These discrepancies could be due to the complexity of a condition where abnormal development is superimposed on the effect of age, and the nature of those interactions is unclear. This is supported by the fact that studies examining relatively young people have also shown differences in brain anatomy and volumes between people with DS and neurotypical people. However, such comparisons do not fully inform on how age interacts with an abnormally developing brain in DS. The effect of age may not be observable until later decades.
Beyond developmental abnormalities, AD-related neurodegeneration may also contribute to macroscopic brain changes in DS that are observable on MRI. For example, synapse and neuron loss in the hippocampus and entorhinal cortex can be measured using volume and cortical thickness [34–36] and suggest a pattern that is comparable to what is observed in sporadic AD. Overall, the extant literature suggests that DS may share both pathological and neuroimaging features of sporadic AD in the general elderly population [35, 37].
Cerebrovascular abnormalities
Accumulating evidence from MRI studies supports the notion that cerebrovascular disease is linked to increased risk of AD in normal aging [38–40]. Vascular lesions, such as WMHs and lacunar infarcts, are linked to late onset AD and cerebral amyloid angiopathy in the neurotypical population and found to be predictive of cognitive decline. Neuroimaging-based markers for cerebrovascular disease implicated in AD, therefore, may be useful for predicting the development and progression of AD in any aging population. While people with DS are at a high risk for developing AD pathology decades prior to onset of dementia, they show low prevalence of vascular risks, such as hypertension, diabetes, dyslipidemia, or smoking [41–44]. Thus, DS represents an important study cohort for investigating the cerebrovascular features of aging and AD in a circumstance of low systemic vascular disease comorbidity [45]. Yet, there has been only a small body of research focused on MRI-defined cerebrovascular lesions in the aging brain of people with DS.
Roth et al. used visual inspection of T2-weighted scans by experienced radiologists and graded the presence and degree of white matter lesions, showing increased prevalence of such lesions in people with DS compared to neurotypical people [46]. Consistent with this, recent work by Lao et al. [47] used T1-weighted and T2 FLAIR images to evaluate white matter vascular pathology in older people with DS who were cognitively stable as well as those with mild cognitive impairment (MCI) or AD. Despite only a few reports of traditional vascular risks (hypertension, diabetes, and hypercholesterolemia), MRI-based cerebrovascular disease markers, including lobar microbleeds, infarcts, and WMHs, were prominent. Specifically, these observations increased with age and with increasing diagnosis severity after adjusting for age. Vascular findings were not strongly associated with the degree of amyloid pathology, particularly after adjustment for their shared association with age. These findings suggest that cerebrovascular disease may be a fundamental feature of AD in people with DS that may have been previously underappreciated. Cerebrovascular pathology as a function of age and AD in DS is expanded upon in Chapter 4.
Longitudinal changes that predict dementia in DS
In DS, early signs of dementia may easily be overlooked as it could be masked by intellectual disability. In this high-risk population, limited information is available with respect to neurodegenerative and cognitive changes during the early stages of AD [48], and longitudinal assessment is essential to identify brain anatomical changes at an early stage and track brain changes with disease progression. This is critical for early and accurate diagnosis.
One longitudinal study performed two brain computed tomography (CT) scans in people with DS (n = 14) at an interval of 10 years, four of whom developed symptoms of cognitive decline [21]. Despite the clear differences in cognition at follow-up, differences in brain atrophy patterns were not found. The authors concluded that age is a better predictor of dementia than imaging, suggesting that cerebral atrophy may be detectable only after substantial cognitive decline has already occurred in DS. However, CT measurements of the brain are crude and do not capture detailed anatomical changes (e.g., focal hippocampal atrophy) that could have otherwise been identified with high-resolution MRI or more sensitive measures.
Interestingly, an earlier MRI study reported similar findings in a small sample of people with DS scanned at two timepoints, noting no significant change over time in the hippocampus, amygdala, or total brain volume in either the demented (n = 6) or nondemented (n = 13) groups [28]. A more recent study showed significant gray matter volume reduction in the basal forebrain, right orbitofrontal cortex, and lateral aspect of the right temporal lobe [49]. Significant white matter volume reduction was also identified in regions implicating the right hippocampus and the left arcuate fasciculus. People with DS who converted to MCI or dementia showed significant gray matter volume reduction in substantia innominata and left hippocampus. In addition, poor memory performance (measured by a version of the Rey Auditory-Verbal Learning Test adapted for people with intellectual disability) was associated with longitudinal atrophy in the left hippocampus and amygdala.
Summary of structural neuroimaging in DS
Structural neuroimaging provides a useful tool for assessment of changes in brain anatomy, morphology, and vascular features in aging people with DS. Despite challenges present for imaging people with DS, consensus findings point to early brain aging and accelerated brain atrophy associated with DS, characterizing this population as remarkably vulnerable to AD neurodegeneration. Structural changes in the brain have been also associated with cognitive decline and AD-like clinical symptoms, suggesting that neuroimaging measures can facilitate early diagnosis of dementia. In addition to observable changes in brain volumes and evidence of cerebrovascular lesions, the structure of circuits connecting across the brains regions that undergo neurodegeneration or are impacted by AD pathology is equally important to understand. Such abnormalities are characterized by diffusion-based neuroimaging, as discussed in the following section.
Diffusion-based neuroimaging
Technical background
Diffusion-weighted imaging (DWI) is a noninvasive technique to characterize the microstructural features of soft fibrous tissues, such as white matter tracts connecting different parts of the brain [50]. It is also useful for detecting changes in brain networks across aging or disease progression [51] and has become a widely used tool for assessment of white matter structural integrity. Diffusion imaging measures are based on the properties of diffusion of water molecules in cellular compartments [52]. Diffusion tensor imaging (DTI) models the diffusion signal in terms of the ‘tensor model,’ which is a measure describing the magnitude and direction of water molecules [53]. The rate and direction of diffusion can be isotropic (equal in all directions) or anisotropic (unequal), which depends on the presence of barriers (e.g., cell membranes, white matter tracts, myelin sheaths) in the brain. Images can be generated as the average of diffusion tensors in three dimensions, generating maps that give information about anisotropy and directionality of water diffusion.
Diffusion anisotropy can be quantified by different metrics. Fractional anisotropy (FA) is most often used to quantify white matter integrity, with values that range from 0 (highly isotropic—poor white matter integrity) to 1 (highly anisotropic—good white matter integrity). Reduction in FA implies loss of tissue integrity, which could stem from axonal degeneration, demyelination, disorganization, packing density, and other microstructural features. Radial diffusivity is thought to be modulated by white matter demyelination, while axial diffusivity appears to be associated with axonal degeneration. However, direct validation of these measures with their underlying neurobiological signatures remains challenging [54]. Anisotropy measures are also subject to thermal or physiologic noise or artifacts (e.g., head motion). A major limitation also is related to the tensor model itself and its inability to resolve crossing, bending, and twisting fibers. These challenges notwithstanding, DTI remains a powerful tool that can help our understanding of the structural connectivity alterations associated with dementia in DS.
White matter integrity in aging and AD in DS
In addition to volume loss, disrupted white matter integrity has been consistently reported in normal aging, with many studies reporting FA decreases in the frontal regions and limbic pathways [55–57]. Disrupted white matter integrity has been associated with AD pathology [58, 59]. WMHs have been related to reduced FA, indicating a potential link between vascular deficits and white matter degeneration [60]. Furthermore, white matter microstructural integrity is associated with memory and executive functioning in nondemented neurotypical older adults [61, 62] and is decreased in amnestic MCI and AD [63, 64]. White matter changes typically observed in AD have also been observed in postmortem studies of people with DS, including evidence of demyelination, microglial activation, and loss of oligodendrocytes and reactive astrocytes. Yet, DTI-based measures of white matter integrity have not been extensively studied in the aging DS population.
Powell et al. observed significantly lower FA in frontal regions in 20 people with DS (10 without dementia and 10 with AD, age range = 35–65) compared to similar aged neurotypical controls [65]. Decreased FA in DS was associated with executive task performance but not with global cognitive status. More reductions in FA in frontal circuits were observed in demented people with DS compared to those without dementia. Fenoll et al. showed in a younger sample of nondemented people with DS (ages 18–52) evidence of reduced FA in frontal-subcortical circuits and increased FA in the temporoparietal junction [66]. Finally, a recent study by Rosas et al. evaluated 51 people with DS (31 cognitively stable, 12 with MCI, and 8 with dementia) using diffusion-weighted MRI and a novel diffusion tractography method for the reconstruction of 18 major cerebral white matter fiber bundles and assessing tract-specific FA as well as axial and radial diffusivity values [67]. People with DS diagnosed with MCI and AD had significant FA reductions (and axial/radial diffusivity increases) in several cortico-cortical association pathways (inferior longitudinal fasciculus, superior longitudinal fasciculus), similar to what has been reported in people with sporadic AD [68]. The consistency of these findings with what has been reported in MCI [69, 70] and in autosomal dominantly inherited AD [71, 72] suggests that the pathophysiology and circuit mechanisms implicated in DS-AD are largely overlapping with sporadic AD.
Summary of diffusion-based neuroimaging in DS
Diffusion-based neuroimaging is an emerging structural MRI technique useful for quantification of changes in white matter connectivity. Similar to gray matter alterations, structural decline in white matter tracts resembles the pattern seen in AD in the general population. Whether these structural changes are related to cognitive decline or dementia remains ambiguous given the limited number of studies and warrants future investigation. Longitudinal DWI studies are also needed for distinguishing structural abnormalities related to dementia in aging DS. Parallel to structural changes, functional changes in network connectivity may also be present in relation to aging and dementia in DS and may serve as earlier markers of decline. The following section describes resting state functional neuroimaging and related studies in aging people with DS.
Resting state functional neuroimaging
Technical background
Functional MRI (fMRI) is a neuroimaging technique that measures brain activity via changes in blood flow, called the hemodynamic response, which depends on the coupling of cerebral blood flow, neuronal activation, and energy consumption by brain cells. As neurons become active, they recruit energy and oxygen from the local blood supply, which leads to a differential in oxygenation level. The difference in the magnetic properties of deoxyhemoglobin and oxyhemoglobin allows for a blood-oxygen-level-dependent (BOLD) contrast to be generated, which can inform on the spatial localization of neural activity. While fMRI is typically coupled with a task, this approach is quite challenging in people with DS. We will focus here on another type of fMRI scan, a resting state scan, which is much more feasible in DS as it does not require task engagement. In 1995 Biswal et al. made an important discovery when they used fMRI to image the brains of people at rest, that is, while they were not explicitly cognitively engaged [73]. In addition to the physiological signals produced by heart rate and respiration, they observed low-frequency (< 0.1 Hz) fluctuations in the spontaneous BOLD signal. Fluctuations in a given region tended to be correlated in time with fluctuations in functionally interrelated brain regions. For instance, left and right sensorimotor cortices showed this temporal synchrony, and this pattern held true for language and visual areas as well. Their discovery suggested that regions of the brain were actively communicating with one another in the absence of conscious engagement [73], in other words, they were intrinsically functionally interconnected.
One particular network of brain regions has been consistently shown to have greater functional connectivity at rest than during cognitive engagement [74], and thus became known as the “default mode network” (DMN) [75]. Initial studies suggested that its component regions include the anterior cingulate cortex, posterior cingulate cortex, medial prefrontal cortex, precuneus, and angular gyrus. Later studies added the medial temporal lobe (MTL) and the retrosplenial cortex in this key network. Studying the DMN in older people and changes to this network in the context of aging and AD has proven quite fruitful.
There are several strengths to using resting state fMRI. It is relatively quick and inexpensive from a research perspective, with a typical resting state fMRI scan lasting only about 10 min. It also has relatively high spatial resolution, with the ability to achieve at least a 1–3 mm resolution scan on a standard 3T scanner available at most neuroimaging research facilities. Much like other MRI-based methods, fMRI is noninvasive, which is important in working with vulnerable patient populations. Resting state fMRI in particular is a simple scan to collect. Participants do not have to learn a task—they are simply asked to lie still in the scanner with their eyes open.
However, there are some limitations to consider when using fMRI. It has low temporal resolution (sampling rate is approximately 1–2 s), which prevents researchers from studying moment-to-moment neuronal activity that takes place at the millisecond scale. Achieving a high signal-to-noise ratio (SNR) is critical for generating usable data, but there are several factors that are known to reduce SNR in fMRI. Certain areas of the brain are subject to signal dropout, such as the rhinal cortex and surrounding medial temporal lobe due to proximity to the sinuses. Additionally, excessive head motion introduces noise, which can confound the data. Some head motion during the scan is inevitable, and fortunately there are ways to account for this added noise after-the-fact using motion correction software. However, certain populations like young children, cognitively impaired older people, and people with DS tend to exhibit higher levels of head movement in the scanner, which significantly compromises the usability of these scans. Depending on the threshold used to censor motion events, anywhere from 7% to 83% of timepoints in an rsfMRI scan from an older adult with DS could be excluded due to excessive motion (unpublished observations). The effect of motion could be mitigated by behavioral training, communication with the participants, and using firm, yet comfortable padding in the head coil reduces the participants’ ability to shift during the scan. However, as mentioned previously, head movement is a significant challenge to imaging in DS.
FMRI, specifically resting state fMRI, has many potential applications for DS-AD research. It can be used to examine functional connectivity differences between demented and nondemented people as well as to track alterations in functional connectivity over time in elderly people with DS. Given its spatial sensitivity it can also be associated with performance on various neuropsychological assessments and may predict cognitive decline longitudinally.
Resting state fMRI alterations associated with aging and AD in DS
There are few studies examining resting state functional connectivity in older people with DS. Vega et al. found greater between-network functional connectivity in people with DS in their 30 s and 40 s compared to age-matched typically developing controls, in the absence of any differences in within-network functional connectivity [76]. Wilson et al. specifically focused on the differences in DMN functional connectivity between people with DS ages 30–55 and age-matched typically developing controls [77]. Compared to controls, people with DS had increased functional connectivity within the DMN and between the DMN and the rest of the brain. This suggests that there are inherent differences in the functional connectivity of people with DS that are likely attributable to atypical development. Additional analyses showed that amyloid-positive (via C11-PiB) people with DS had decreased DMN connectivity compared to amyloid-negative people with DS suggesting that reductions in DMN connectivity may be a hallmark feature of AD pathology in DS that is not intrinsic to DS-related developmental changes. Finally, a recent study by DiProspero et al. followed older people with DS for two years to track phenoconversion and demonstrated that reduced anterior-to-posterior long-range DMN connectivity predicted conversion to dementia, while local DMN connectivity appeared unchanged [78]. To date, however, there have been no studies looking at longitudinal changes in functional connectivity in people with DS. Such studies are needed to determine whether changes in functional connectivity predict dementia in this population.
A major challenge for functional MRI is that there are inherent differences in cerebral blood flow and blood volume across people, reflecting possible differences in cerebrovascular coupling due to age, genetic conditions, etc. These differences are reflected in fMRI findings and may confound interpretation of results. One way to avoid this problem is by using arterial spin labeling, which allows for a dissection of these contributing factors. The next section will highlight the emerging work using arterial spin labeling in DS.
Arterial spin labeling
Technical background
Arterial spin labeling (ASL) is a neuroimaging technique that quantitatively measures tissue perfusion, or cerebral blood flow (CBF), in units of mL/g/min. It does this by magnetically labeling arterial blood water, which serves as an endogenous tracer, using a radiofrequency pulse on a standard MRI scanner. Cerebral perfusion is thought to reflect cerebral metabolism. ASL does not rely on exogenous contrast agents, like gadolinium, thereby reducing participant exposure to radiation, which is critically important when working with a sensitive patient population like people with DS. However, very few studies have employed ASL to study aging and neurodegenerative disease in DS. Recent research suggests that aging reduces CBF in people with DS, and presence of severe residual arterial signal is associated with dementia in this population [79].
There is high concordance between cerebral metabolism and cerebral perfusion. Studies have demonstrated near-identical brain maps created by FDG-PET and ASL [80]. Given this overlap, ASL can be considered a shorter, noninvasive alternative to FDG-PET. ASL acquisition generally lasts five to ten minutes, whereas an FDG-PET scan session can last more than an hour due to tracer uptake time. ASL measures perfusion without the ionizing radioactively labeled isotope required by FDG-PET by utilizing a magnetically labeled endogenous tracer. Replacing FDG-PET with ASL enables more targeted PET scans for amyloid-beta and hyperphosphorylated tau proteins. Taken together, these benefits make ASL an ideal candidate for studying perfusion in people with DS.
ASL is not without its limitations, however. ASL has poor temporal resolution due to the need to wait for the magnetic label to decay from the blood. Additionally, the signal-to-noise-ratio (SNR) for ASL is low since the signal derived from the subtraction of the tag and control images represents only 0.5%–1.5% of the entire tissue signal. It is also important to note that after age 30, gray matter perfusion gradually declines. Therefore, researchers and clinicians must consider age-dependent reductions in perfusion signal intensity when collecting ASL data in older adults.
ASL changes associated with aging and AD in DS
There is only one published study investigating ASL perfusion in older adults with DS. Thalman et al. found lower global CBF among people with DS who has probable AD compared to nondemented people with DS and nondemented neurotypical controls [81]. Within DS, decreased global CBF was associated with worse cognitive performance. There was also a nonlinear effect of age, wherein global CBF declined more rapidly after 45 years of age. Across all participants, higher severe residual arterial signal scores, which indicate the extent to which the ASL signal is retained in the large arteries of the brain, were associated with decreased global CBF.
Going forward, more regional, hypothesis-driven ASL studies are needed to understand how age- and AD-related perfusion changes in DS relate to changes observed in neurotypical people. Studies in patients with MCI and AD have shown hypoperfusion in temporal and parietal regions. They have also shown that precuneus hypoperfusion may predict AD conversion. Confirming these findings in older people with DS will underscore the predictive value that ASL has for identifying loss of CBF that may contribute to early cognitive decline across clinical populations at risk for AD.
While fMRI and ASL are helpful techniques used to understand brain function and metabolism, respectively, they operate on a relatively macrostructural scale. Understanding molecular changes that may be taking place in the brains of people with DS is also crucial. Magnetic resonance spectroscopy (MRS) captures the chemical composition of brain tissues, measuring the relative amounts of metabolites and metabolic intermediates that are relevant to brain functions known to change in aging and neurodegenerative disease.
Magnetic resonance spectroscopy
Technical background
Proton magnetic resonance spectroscopy (1H MRS) is a neuroimaging technique that quantifies the relative concentrations of various metabolites in the brain using the signal emitted from hydrogen protons. It is typically acquired on the same kind of MRI scanner used to acquire structural, functional, and diffusion MRI images. Using a series of radiofrequency pulses applied to a small region in the brain, it generates a spectrum of metabolites based on their chemical shift. The relative concentration of each metabolite is depicted as the peak wave height at each point on the horizontal axis. Because the ratio of water to metabolites in the brain is roughly 10,000:1, the presence of water effectively washes out the metabolite signal. To correct this, the water signal is suppressed so that the peaks of the metabolites can be distinguished in the resulting spectrum.
More than 80 metabolites can be studied using 1H MRS, but the most commonly studied ones are N-acetyl aspartate (NAA), myo-inositol (MI), choline (Cho), creatine (Cr), and the sum of glutamate and glutamine (Glx) since the two cannot be reliably distinguished on the spectrum (see Table 1). NAA is a marker for neuronal health, specifically mitochondrial function and neuronal density. NAA does not seem affected by normal aging, though low NAA can reflect neuronal loss or injury resulting from neurodegenerative disease. MI is a marker of glial function and is increased in normal aging, perhaps indicating neuroinflammation due to activated astrocytes and microglia. Cho is thought to be a marker of cell membrane integrity. Cho concentration increases with age, which is correlated with increased membrane turnover or cell density. Changes in Cho level may reflect the presence of gliosis, myelination, or inflammation. Cr is important for maintaining brain homeostasis, energy storage, and second messenger signaling. Aging is associated with increased Cr, which may indicate abnormal cell metabolism. Glx is a marker for the excitatory neurotransmitter, glutamate, and the inhibitory neurotransmitter, gamma-amino butyric acid (GABA). Glx tends to decrease with age, which may reflect a change in the balance between glutamate and GABA signaling [88–90].
Table 1
Metabolite | Marker for | Age-related change | Findings in DS |
---|---|---|---|
NAA | Neuronal health (e.g., mitochondrial function and neuronal density) | No change with normal aging, but decreased in neurodegenerative disease | [82–84] |
MI | Glia | Increased in normal aging, possibly as a sign of neuroinflammation via activated astrocytes and microglia | [82–85] |
Cho | Cell membrane integrity | Increased in normal aging related to increased membrane turnover, increased cell density, or presence of gliosis, myelination, or inflammation | [86] |
Cr | Homeostasis, energy storage, and second messenger signaling | Increased in normal aging, possible due to abnormal cell metabolism | None |
Glx | Neurotransmitters (e.g., glutamate, GABA) | Decreased in normal aging, reflecting shift in balance between excitatory and inhibitory transmission, though changes are region specific | [84, 87] |
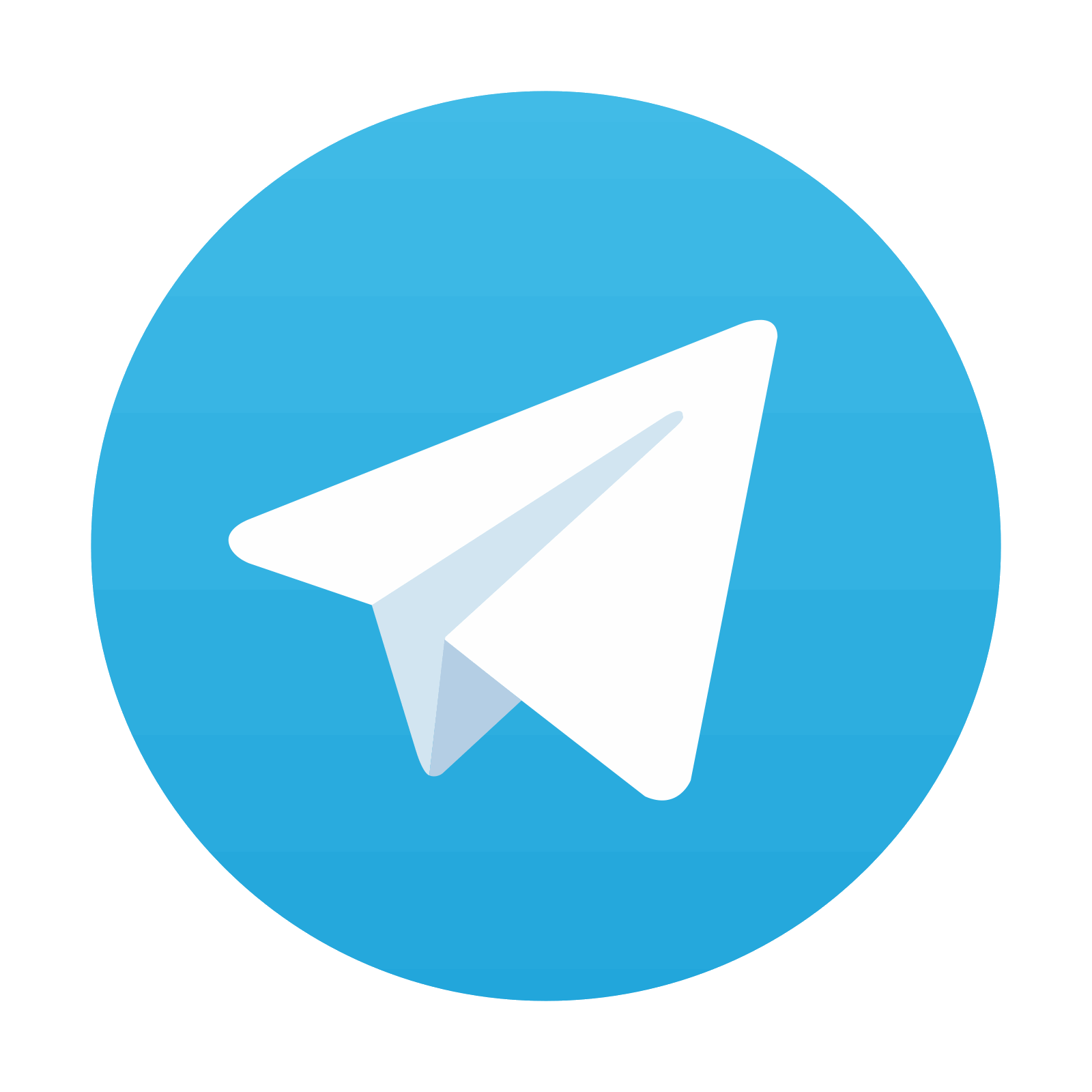
Stay updated, free articles. Join our Telegram channel
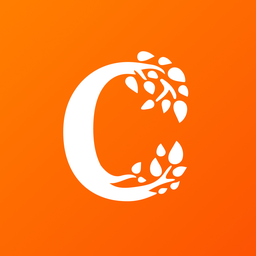
Full access? Get Clinical Tree
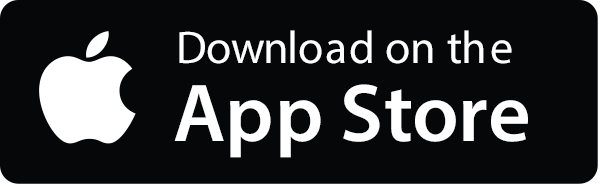
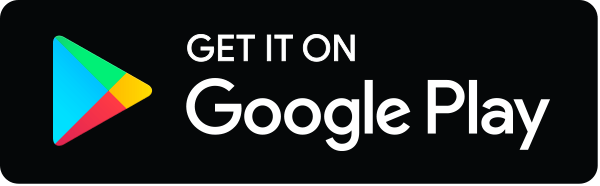