Microneurography was developed by Karl-Erik Hagbarth and Åke Vallbo in Uppsala, Sweden in the early 1960s, and the first definitive papers were published in 1968 (on cutaneous and muscle afferents). With this invasive technique it is possible to study how receptors in muscle, skin, and joints behave under natural conditions and how the sympathetic innervation of skin and muscle normally behaves. A number of authoritative reviews of the technique and the information obtained thereby have been published.
Microneurography has generated many data that illuminate normal neural mechanisms and the disorders that occur in a wide variety of disease processes. The development of conduction block in single axons has been studied in microneurographic recordings, as has the ectopic activity responsible for paresthesias. It is also possible, for example, to record compound sensory action potentials containing identifiable deflections due to large and small, myelinated and unmyelinated axons. However, this can be done almost as readily and less invasively using near-nerve needle recordings.
Overall, microneurography seems to be of limited diagnostic value. There are four main reasons. First, it takes skill and perseverance to obtain satisfactory recordings. Second, only a few satisfactory recordings from single axons can be obtained in any one session, too few for meaningful statistical comparisons against control values while, with population responses involving many axons, the recorded activity varies with the position of the microelectrode within the nerve fascicle. As a result, the intensity of neural activity can vary greatly from experiment to experiment. Third, if pathology leads to a loss of activity (rather than activity that is preserved but abnormal), as in autonomic failure, no activity will be recorded. This then begs the question of whether the absence of activity is due to technical failure or the pathologic process. Finally, the trauma of the study is a consideration, not because of discomfort, but because, conceivably, the microelectrode could damage the few surviving axons in a patient with, for example, amyotrophic lateral sclerosis, or exacerbate the pain of a patient with a complex pain syndrome.
While microneurography has resulted in major changes in thought about the nature of the feedback that the nervous system receives from peripheral receptors and how it uses that feedback for perception and for movement, this chapter will focus on findings that shed light on clinical phenomena. The reader is referred to previously published reviews for more comprehensive treatises on normal physiology.
Recording technique
The basic technique has changed little since first described. Most authorities use a microelectrode made from tungsten with a shaft diameter of approximately 200 μm, insulated to the tip. The greater the diameter, the more that penetration of a fascicle hurts; a diameter of less than 200 μm is not sufficiently rigid. The experimenter inserts the microelectrode manually through the skin to impale the peripheral nerve. Commonly, weak electrical stimuli are delivered through the electrode and the experimenter hones in on an appropriate nerve fascicle, guided by the evoked paresthesias (when seeking a cutaneous fascicle) or the twitch contraction (when seeking a motor fascicle). Vallbo and colleagues initially insert a low-impedance electrode to locate an appropriate fascicle and then use its location to guide the insertion of the recording microelectrode, the impedance of which has been decreased to near-optimal levels. Others rely on the passage through the skin and the subcutaneous and perineural tissue to remove insulation and reduce the impedance to a satisfactory level. When in place, the optimal impedance of the electrode is perhaps 50 kΩ for multi-unit recordings and some 100 to 150 kΩ for single-unit recordings (though higher impedances may assist single-unit C fiber recordings).
Once the experimenter has manipulated the tip into the fascicle, afferents in that fascicle can be activated, for example by tapping on the muscle tendon or scraping the skin ( Fig. 14-1 ). The activation of mechanoreceptors creates an auditory signal that can be used to guide further small adjustments of the electrode. The electrode is not fixed securely because external fixation leads to electrode dislodgement when minor movements inevitably occur. It is stabilized at its proximal end by a coiled insulated copper wire to the preamplifier and at the recording end by the rigidity of the tissue into which the electrode has been inserted. Nevertheless, minor movements—particularly those that displace skin at the recording site—can jeopardize the recording. This creates an issue for motor control studies because the range of movement that can be studied is small, and consequently it is prudent not to generalize too far about, for example, the role of the fusimotor system in normal motor behavior (see below).

The insulation created by the tissue that surrounds a nerve fascicle is very effective. It is virtually impossible to record the activity of single axons if the microelectrode tip is just outside a fascicle, even if the discharge of that axon is used to trigger averages of many sweeps. Once the microelectrode is within a fascicle, axonal activity can be recorded readily, and it is then possible for the experimenter to focus on spontaneous neural discharges (e.g., the bursts of activity that occur in sympathetic efferent axons innervating skin or muscle) or the neural discharge evoked by mechanical stimuli, such as tapping on the muscle belly or tendon (with a motor fascicle) or stroking the skin (with a cutaneous fascicle).
Obtaining stable recordings from single axons requires skill and patience. It is helpful if the axon has a background discharge because, unless the discharge of the axon can be heard, it may be difficult to know that the electrode is close to it. This means that the recordings inevitably will be biased toward axons that maintain a background discharge, and this is probably the main reason that there are fewer recordings from group Ib afferents from Golgi tendon organs than from group Ia afferents from primary spindle endings. In addition, because action potential amplitude is a function of the cross-sectional area of the axon, the potentials of large axons will be distinguished more readily from the background noise—perhaps explaining why there are few recordings from cutaneous Aδ axons. Paradoxically, it is relatively easy to obtain recordings from unmyelinated C fibers. This is because they occur in clusters of approximately six or more axons within the cytoplasm of a Schwann cell (the so-called Remak bundle) containing C fibers with different properties, so that a recording from this group of axons can be made quite readily, particularly if they are sympathetic and have a background discharge. The action potentials from myelinated axons commonly have a bifid appearance (in about 75 percent of units, Fig. 14-2, C ). The two peaks represent action potentials at nodes of Ranvier on either side of the impalement of the axon by the microelectrode ( Fig. 14-2, D ). That two peaks are discernible implies that conduction across the internode is slow (see later, in section on Conduction Block). When the action potential has a simple biphasic morphology (as in Fig. 14-2, A ), it could be “normal” or one in which conduction across the impaled internode is blocked.

The technique has been developed to allow stimulation through the intrafascicular microelectrode (often referred to as microstimulation ). With myelinated axons, activation is likely to occur at the nodes of Ranvier on either side of impalement (as in Fig. 14-2, C and D ). In studies of the perceptual correlates of afferents, this effectively then would be the proximal node because any action potential from the distal node will occur during the refractory period for the proximal node, even when internodal conduction is delayed (see later). With afferents, intrafascicular stimulation has allowed (1) the cortical evoked potentials produced by the stimulated afferents or their reflex effects to be defined, and (2) the perceptual responses associated with activity in single afferent axons to be documented. With efferents, intrafascicular stimulation has been used (1) to produce the twitch contraction of the receptor-bearing muscle to differentiate spindle endings from Golgi tendon organs, as discussed later, and (2) to document the contraction properties of single motor units.
The critical step with single units is to identify the axon. This can be done for afferents by locating the receptor in skin or muscle and characterizing its responses to a variety of stimuli: mechanical, thermal, nociceptive. With α motor axons, the axon will be active only if there is a deliberate, inadvertent, or involuntary contraction of the innervated muscle involving that motor unit, and the neural spike can then be used to spike-trigger average the ongoing electromyographic activity ( Fig. 14-3 ). This then should allow the motor unit action potential to be defined at a latency appropriate for direct conduction from the stimulating site to the muscle. It has been claimed that γ motor axons (which innervate the intrafusal muscle of the muscle spindle) can be identified on discharge characteristics alone, but it would be prudent to retain reservations about such claims because there are scant data on how γ motor neurons discharge in natural acts in animals, and no such data for human subjects. Accurate identification can be time-consuming, and may require maneuvers that displace the skin at the recording site (e.g., a twitch contraction), so that recordings are lost before meaningful data can be obtained from that axon.

Muscle afferents
The motor control literature has focused on large myelinated axons innervating the muscle spindle and the Golgi tendon organ, which are well-defined encapsulated receptors. Such axons are termed group I (Ia afferents from the primary ending of the muscle spindle; Ib from the Golgi tendon organ) or group II (slightly smaller axons from the secondary ending of the muscle spindle and also from nonspindle receptors). Given conduction velocities of approximately 60 m/sec for human group I afferents and approximately 40 m/sec for human group II afferents, the diameter of group II afferents can be estimated as 9.8 to 16 μm if group I axons have diameters of 12 to 20 μm. However, there are many more afferents with small myelinated or unmyelinated axons that arise as free nerve endings, and they are responsive to mechanical stresses, and chemical, thermal, and nociceptive stimuli. There have been only a few studies on slowly conducting human muscle afferents and their correlation with muscle pain, and they will not be addressed further.
The primary ending of the muscle spindle is exquisitely sensitive to small perturbations, arguably more so than any sensor that we can devise to detect the perturbation. Characteristically, the endings are said to respond to the extent of stretch and to its velocity ( Fig. 14-4, B ), and their responsiveness can be altered by producing (nonpropagated) contractions of the modified muscle fibers (“intrafusal fibers”, i.e., muscle fibers within the fusiform muscle spindle) which they encircle. These focal contractions are produced by γ motor axons (which innervate only the muscle spindle) and/or β motor axons (which innervate both the muscle spindle and nearby muscle fibers, so-called “extrafusal” muscle). Two types of γ efferent are recognized: those with predominantly “dynamic” effects on the discharge of the primary ending and those with predominantly “static” effects.

The secondary ending of the muscle spindle has little sensitivity to the derivatives of length, and it operates more as a length transducer (see Fig. 14-4, B ; 14-5, D ). Each spindle gives rise to a number of group II afferents but only a single group Ia afferent. Only static γ motor axons will affect the discharge of the secondary ending.

The muscle spindle lies in parallel with surrounding muscle fibers so that their contraction will shorten the spindle and relaxation will stretch it. However, some muscle fibers insert directly into the capsule of the spindle and there may be fascial interconnections between spindle and muscle fibers, so that contraction of the relevant motor units may activate spindle endings directly rather than unload them. Primary and secondary endings on the muscle spindle behave similarly, with the exception that any change in discharge of the primary ending contains a dynamic component. The term “Golgi tendon organ” is a misnomer because few tendon organs lie within the tendon itself. Tendon organs occur where muscle fibers insert into fascia or tendon and, in most muscles, tendon organs are located mainly within the anatomic confines of the muscle belly. Between 5 and 15 muscle fibers insert into the capsule of any one tendon organ, and these fibers generally come from different motor units, so a single tendon organ samples the activity of a subset of motor units but only those that are anatomically close. These receptors are exquisitely sensitive to force applied directly to them, and the contraction of a single muscle fiber may be sufficient if it inserts into the tendon organ capsule. Under isometric conditions, the summed activity of a number of tendon organs accurately reflects overall contraction force. Tendon organs are, however, much less sensitive to the forces produced by muscle stretch, even though they are grouped together with spindle endings as “stretch receptors.” In noncontracting muscles, tendon organs usually have no background discharge and, given that most microneurographic studies are performed with the subjects initially at rest, it is not surprising that the responses of tendon organs are less well documented than those of muscle spindles.
The differentiation of Ia, II, and Ib afferents depends on the use of a twitch test to distinguish a spindle ending from a tendon organ, the variability of any discharge that is present at rest (spindle endings only), and the responses of the ending to a variety of stimuli (stretch and shortening, contraction, vibration). The most popular methods for producing the twitch contraction involve stimulation of the innervating nerve trunk or stimulating maximally within the selected motor fascicle. The former will produce a larger twitch contraction, thereby risking dislodging the microelectrode, and may activate other muscles so that the twitch contraction is not confined to the receptor-bearing muscle, risking that the afferent might be unloaded by a dominant contraction of an unintended muscle. The latter method restricts the contraction to the receptor-bearing muscle, but requires a special amplifier which can be switched off transiently to allow the stimulus to be delivered through the microelectrode and then switched back on, with a gap of only some tens of milliseconds or less. A third method involves stimulating through surface or needle electrodes inserted over or into the receptor-bearing muscle, surface electrodes being suitable only with superficial muscles.
The typical unloading/reloading (“in-parallel”) responses of muscle spindle endings in the twitch test are shown in Figures 14-4, A and 14-5, E . Typically the primary ending may resume firing on the falling phase of the twitch contraction with a high-frequency burst of impulses (see Fig. 14-4, A ). By contrast, tendon organs are activated on the rising phase of the twitch, and this produces a loading (“in-series”) response. With muscle stretch and shortening, the Ib response is minimal, that of Ia afferents characterized by a dynamic response to the movement with a steady discharge at an adapted level to maintained stretch, and a pause in discharge on shortening (see Fig. 14-4, B ). Typically, group II afferents lack the dynamic response to stretch and the pause on shortening. Dependent on muscle length, many spindle afferents and all Ib afferents are silent in relaxed EMG-silent muscles, but for those that are active, the variability of discharge of Ia afferents is higher than that of group II afferents. Both tendon organs and muscle spindles may be activated during a voluntary contraction, the former stimulated directly by the muscle contraction, the latter because fusimotor neurons are activated along with α motor axons by voluntary effort.
Clinically Relevant Insights
Recordings from muscle spindle afferents can provide insight into the role of the γ efferent (fusimotor) system. However, this insight is quite indirect, and only as good as the controls used to ensure that any change in spindle behavior is not due to inadvertent stretch or contraction of the receptor-bearing muscle. Using rigid controls creates a dilemma because the constraints may well change the subject’s mental set and thereby the way in which fusimotor neurons behave. In addition, the risk of jeopardizing recordings limits the types of movement that can be studied. It would be surprising if the recordings so far reported for humans are truly representative of the full motor repertoire at our disposal but, even allowing for this, it is clear that the human fusimotor system behaves differently from that of the awake behaving cat. This should not be surprising, given that there are major species differences: size, length of conduction pathways, conduction velocities, morphology of spindle endings, and differences in motor skill. For example, the quadrupedal digitigrade stance and locomotion of the cat differ from the more unstable bipedal plantigrade stance and locomotion of humans, in whom there seems to be greater reliance on anticipatory and feedforward control mechanisms than on feedback adjustments.
If one accepts these reservations, recordings from spindle afferents do allow a number of conclusions to be put forward.
Sensory Role of Muscle Spindles
Microstimulation of single spindle afferents produces no discernible sensation, but activation of a population of afferents by electrical stimulation or tendon vibration can evoke sensations of movement or a change in position. These illusory movements can be quite strong, sufficient to move a limb or joint to an anatomically impossible position. However, muscle afferents are not the sole source of proprioceptive cues, and the responses to microstimulation are not unlike those with stimulation of cutaneous SA2 afferents (see below).
Fusimotor Function at Rest
There seems to be general agreement that the level of γ s drive (i.e., drive from γ-static neurons) directed to noncontracting muscles is low, if it exists at all—certainly too low to have a significant effect on spindle function. The extent to which this applies to γ d activity (i.e., activity of γ-dynamic neurons) is uncertain. There have been intermittent reports of spindles behaving as if they do receive some background γ d drive, even in relaxed muscles, but nevertheless any such background drive is not a prerequisite for a tendon jerk. De-efferenting spindles by injection of local anesthetic around the nerve does not alter their responses to changes in muscle length ( Fig. 14-6 ) or their responses to tendon percussion.
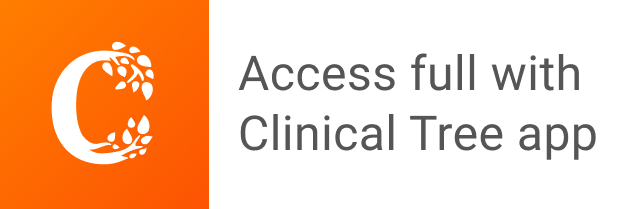