Nerve conduction studies assist in the evaluation of neuromuscular diseases by providing a physiologic assessment of the peripheral nerve, muscle, neuromuscular junction, dorsal root ganglion cell, and anterior horn cell. They provide the greatest help in assessing peripheral nerve disease. Motor nerve conduction studies assess motor axons by selectively recording muscle responses to nerve stimulation. Sensory nerve conduction studies assess sensory axons by stimulating or recording from peripheral nerves with predominantly sensory axons. Nerve conduction studies most often confirm a clinical diagnosis, but they are also valuable in:
- 1.
Excluding other suspected disorders
- 2.
Identifying unrecognized (subclinical) disorders
- 3.
Localizing focal abnormalities along a nerve
- 4.
Defining severity with objective measurements
- 5.
Characterizing abnormalities, such as conduction block or demyelination
- 6.
Helping to distinguish axonal disorders from anterior horn cell, neuromuscular junction, muscle, and central disorders, and
- 7.
Identifying anomalous innervation.
Motor nerve conduction studies were first described for clinical use in 1948. Subsequent studies defined normal values and the abnormalities seen in clinical disorders. Motor nerve conduction studies have expanded steadily in application since then. Sensory nerve conduction studies initially were demonstrated experimentally by Dawson and Scott in 1949, and were shown to be of clinical value in 1958 by Gilliatt and Sears. Sensory nerve action potentials (SNAPs) are of much lower amplitude than compound muscle action potentials (CMAPs) and are sometimes below the level of noise and artifact in the recordings. Averaging of multiple potentials is often required to record them reliably, particularly at proximal locations.
Motor nerve conduction studies
The motor axons of any peripheral nerve that innervates somatic muscle can be evaluated by motor nerve conduction studies if the nerve can be stimulated and if the response of one or more muscles innervated by that nerve can be recorded electrically. Standard studies of motor nerve conduction assess the most accessible nerves (i.e., the ulnar and median nerves in the upper extremities and the tibial and fibular [peroneal] nerves in the lower extremities). Reliable motor nerve conduction studies also can be performed on the musculocutaneous, radial, facial, spinal accessory, axillary, suprascapular, femoral, phrenic and spinal nerves, but with increasing technical difficulty.
Stimulation of any of these nerves evokes both an electrical and a mechanical response in the muscles innervated by the nerve distal to the site of stimulation. The mechanical response, or muscle twitch, is not recorded in standard clinical nerve conduction studies, but it may be measured for constructing strength–duration curves or for other special studies. The electrical response is called the compound muscle action potential ( Fig. 13-1 ). It is the summated electrical activity of the muscle fibers that are in the region of the recording electrode and that are innervated by the nerve. The general techniques of stimulating and recording are similar for all motor nerves.

Stimulation
Nerve stimulation is achieved most readily with surface electrodes placed over a nerve where it is relatively superficial, such as over the ulnar nerve at the wrist and elbow. If there is overlying edema or fatty tissue, or if the nerve is deep, a needle electrode permits more precise stimulation with a lower voltage, with only minimal risk of trauma to neighboring structures.
The standard stimulus is a square-wave pulse of current passing between two poles; the cathode (−) depolarizes the underlying axons, while the anode (+) hyperpolarizes them. Usually, the cathode is closer to the recording electrodes than is the anode to prevent block of axon conduction by hyperpolarization. A gradually increasing stimulus voltage produces a progressive increase in the size of the CMAP as more and more axons in the nerve are activated; the stimulus is increased by 30 percent after the CMAP no longer increases (i.e., the stimulus is supramaximal) to ensure activation of all the motor fibers. A stimulus of 25 mA (100 V) and 0.1 msec in duration is usually sufficient to activate all the large myelinated fibers in a nerve. Although large, low-threshold, fast-conducting axons should be stimulated initially, variations in the location of nerve fascicles and in the patterns of current flow may result in the initial activation of smaller axons with slower conduction. However, for abnormal nerves or for deep nerves, the stimulus may need to be as much as 75 mA (300 V) and 0.5 msec in duration, and it may need to be delivered with a monopolar stimulator (distant anode) or with a near-nerve needle electrode. Nevertheless, it must be remembered that large stimuli may produce errors by stimulating other nearby nerves inadvertently or by activating the nerve at a distance from the stimulating electrode.
Magnetic stimulation of peripheral motor nerves can be less painful and has been suggested as an alternative to electrical stimulation for nerve conduction studies. However, the site of stimulation cannot be defined well enough to permit reliable calculation of velocity, and maximal responses cannot be obtained reliably in diseased nerves. Magnetic stimulation at the spinal nerve level does not produce maximal activation of nerve fibers.
Recording
The CMAP is recorded with a pair of electrodes placed over a muscle or group of muscles innervated by the nerve. Recordings are often made from only a subset of all the muscles innervated by the nerve; thus, the recordings assess only the axons innervating those muscles. For instance, recording from the hypothenar muscles is used commonly in tests of ulnar nerve motor conduction. Selective damage to the axons that innervate either the flexor carpi ulnaris muscle or the first dorsal interosseous muscle therefore would not be identified unless recordings were made also from those muscles. Recent studies have shown that recordings from the first dorsal interosseous and the flexor carpi ulnaris muscles can increase the identification of ulnar neuropathies by up to 20 percent. Recording from anterior compartment muscles when no response is obtained distally with fibular (peroneal) nerve stimulation can provide valuable information as well.
Standard anatomic locations of recording electrodes provide reproducible potentials that can be compared with normal values. Measurements of a CMAP are most reliable when recordings are made over the endplate region of the muscle, near the middle of the muscle belly. Recordings are made with a bipolar derivation: an active (G1) electrode over the endplate region and a reference (G2) electrode over the tendon. Larger electrodes result in smaller CMAPs but give greater reproducibility.
Recordings may be made with surface or needle electrodes. Surface electrodes provide the advantage of nontraumatic recordings with less risk of acquired immunodeficiency syndrome (AIDS), hepatitis, and Creutzfeldt–Jakob disease. They can be repositioned more readily to obtain the maximal, initially negative response. Needle electrodes have somewhat higher impedance but can be placed in the subcutaneous tissue immediately adjacent to the muscle to provide a more accurate reflection of the size of the CMAP than do surface electrodes, which may be some distance from deep muscles. Needle electrodes placed within muscle are less reliable because of an unstable configuration and amplitude of the CMAP during muscle movement. However, intramuscular electrodes can record from a more limited area of the muscle and may be of value in assessing individual motor branches of a nerve, atrophic muscle, and anomalous patterns of innervation.
Measurements
The directly evoked CMAP recorded after stimulation of a peripheral nerve is called an M wave. With supramaximal stimulation, all the fibers in a muscle innervated by the nerve contribute to the potential. The earliest component is elicited by the fastest-conducting motor axons. The M wave usually is described by its latency, amplitude, area, and configuration.
Latency is the time in milliseconds from the application of a stimulus to the initial deflection from the baseline, either positive or negative, and is the time required for the action potentials in the fastest-conducting fibers to reach the nerve terminals and activate the muscle fibers ( Fig. 13-2 ). Since the latency of the peak of the CMAP represents activation of different axons at proximal and distal sites of stimulation, peak-latency measurements are not reliable for determining motor conduction velocity When recording is done over the endplate region of the muscle, the normal configuration of the CMAP is biphasic, negative–positive. If the active electrode is not over the endplate region, the potential is triphasic, with an initial positivity that makes latency measurements less accurate. Since the distal latency varies directly with the distance between the stimulating electrode and the muscle, a fixed distal distance improves reliability by eliminating distance differences among individuals.

Normally, only minimal changes in configuration occur with stimulation at proximal sites compared to the responses recorded at distal sites. In some recordings, particularly fibular nerve conduction to the extensor digitorum brevis (EDB), an initial positivity is seen with proximal, but not distal stimulation because of volume conduction of the CMAP from the distal portion of the extensor hallucis longus muscle. The positive component must be ignored in measuring latency since it is not from the EDB. In this case, latency should be measured to where the negative upslope of the CMAP crosses the baseline to become negative.
The distal latency from the most distal site of stimulation includes slowing in the smaller nerve terminals and the neuromuscular transmission time. Thus a conduction velocity cannot be calculated from the distal latency alone. However, an alternate calculation of “residual latency” that compares the expected latency (based on proximal conduction velocity) with the actual latency may be of value in identifying distal demyelinating processes.
If the nerve is accessible, it is stimulated at more than one site along its course to evoke two or more CMAPs from the muscle. The latencies, areas, amplitudes, and configurations of the CMAP at each site are compared. Measurement of the differences in distance and latency between sites of stimulation allows conduction velocity calculation in the segment of nerve between the sites of stimulation in meters per second. Accurate distance measurement is of critical importance in calculating velocity. Longer distances improve the reliability of velocity calculation.
Comparison of conduction in selected segments with that in other segments of the nerve and with normal values can localize a lesion along the length of a nerve. Normal conduction velocities are from 40 to 55 m/sec in the legs and from 50 to 70 m/sec in the arms. Conduction is faster in proximal segments of nerves, thus resulting in an inverse relationship between body height and conduction velocity.
With supramaximal stimulation, the area (in msec.mV) of the negative phase of the action potential measures the number of muscle fibers depolarized and shows only minor change with the distance of the muscle from the stimulating electrodes. Change in CMAP area is the most reliable measure of loss of functioning axons between two points along a nerve and should be measured if the recording equipment permits. Because the contribution of individual motor units to the CMAP is determined by the location of the recording electrodes, they should be applied in standard locations.
If area cannot be measured, amplitude can serve the same purpose, provided that the duration of the CMAP does not change between stimulation sites. The amplitude of the CMAP usually is measured as the height in millivolts from the baseline to the peak of the negative phase. The amplitude normally is between 2 and 20 mV, but is somewhat lower in some nerves when more proximal sites are stimulated. The area and amplitude of the CMAP should be measured routinely because they are proportional to the number of muscle fibers activated and provide an estimate of the amount of functioning nerve and muscle. The area and amplitude of the CMAP are affected by a number of variables. The distance between the recording electrode and the muscle has the greatest effect; thus, the presence of edema or excess fat results in CMAPs with small areas and low amplitudes. Fortunately, edema and fatty tissue are recognized easily, so that the low amplitudes are not mistaken for signs of disease.
The area, amplitude, duration, and latency of the CMAP are dependent on a number of factors that must be considered when nerve conduction studies are quantitated. The number of activated axons directly affects the size and shape of the CMAP. For consistency of measurement, supramaximal responses are recorded in standard nerve conduction studies. Submaximal responses have longer latencies, smaller areas, and lower amplitudes. To obtain the maximal response with no initial positivity, the active electrode should be over the endplate region of the main bulk of the muscles innervated by the nerve being tested, with the reference electrode over the tendon of the muscle. The interelectrode distance between the active and the reference electrodes directly affects the area and amplitude of the CMAP and must be standard for each nerve. It must be greater for longer muscles to allow placement of the electrodes over the endplate region and tendon. The CMAP differs when a subcutaneous or an intramuscular needle electrode is used in place of surface electrodes. The temperature of the limb and muscle also affects the CMAP; larger areas, higher amplitudes, and longer latencies are found with lower temperatures.
The amplitude and latency of the CMAP change with the site of stimulation. At more proximal sites, the latency increases progressively with increase in distance that the action potential has to travel to the muscle. The amplitude decreases as the synchrony of discharge of the contributing motor units decreases. If all axons conducted at the same velocity, the amplitude would remain unchanged; but because they differ, slower-conducting axons activate the muscle progressively later with more proximal stimulation so that the CMAP becomes longer and lower. Partial CMAP cancellation of negative components by overlapping positive components results in only minimal reduction in area compared to amplitude at more proximal sites of stimulation. A reduction in area or amplitude at a proximal site compared to a distal site of stimulation that is greater than that of a normal nerve is evidence of disease. This reduction can result from diffuse disease with a gradual reduction along the length of the nerve. In contrast, a focal lesion may result in a CMAP reduction over a short distance, called a conduction block. A conduction block is one of the most reliable signs of an acute compression neuropathy. The extent of normal reduction in area and amplitude with more proximal stimulation varies from nerve to nerve; values in individual cases must be compared with normal values for that nerve. In most limb nerves, the reduction in area and amplitude with stimulation between the wrist or ankle and elbow or knee is less than 20 percent.
The duration of the response is the time, measured in milliseconds, from the onset to the end of the negative phase of the M wave. Differences in conduction velocity result in the nerve action potentials generated by a single stimulus reaching the muscle slightly dispersed in time. The duration normally increases slightly at more proximal sites of stimulation compared to distal sites. Greater variation in axon velocities results in greater CMAP dispersion with a longer duration. The change in duration of the response provides an approximation of the range of conduction velocities in motor axons. Other methods have been proposed to measure conduction in slower fibers. Paired stimulation (collision) is significantly more time-consuming and seldom provides useful clinical information. F-wave methods can demonstrate more dispersion because of the longer course of nerve traversed (see Chapter 18 ).
If the action potentials of slower-conducting motor units spread out by dispersion, the CMAP breaks down into an irregular waveform with spike components. This occurs with the pronounced slowing of conduction velocity in demyelinating disorders and with reinnervation. Dispersion of the CMAP can provide evidence of underlying disease even when the fast-conducting fibers still conduct at normal rates. The most reliable quantitation of a dispersed CMAP is the duration of the negative phase of the CMAP. The increase in duration at proximal sites of stimulation differs for different nerves. Normal values must be defined for each nerve. Identifying the end of the positive phase of the CMAP is more difficult, especially in disease with complex CMAPs having multiple baseline crossings and phases. The duration of such waveforms is best measured to the last baseline crossing.
The collision and F-wave latency methods have been reported to measure conduction of slower motor fibers by stimulation of the nerve at proximal and distal sites. In the collision method, two stimuli are applied simultaneously, one at a proximal and the other at a distal site on the nerve. The antidromic CMAP (going up the nerve) from the distal site collides with and cancels the orthodromic (down the nerve) CMAP from the proximal site, so that only the orthodromic CMAP of the distal stimulation is recorded. If the interval between the time of stimulation of the distal and proximal sites is increased gradually, at a certain interval only the more slowly conducting fibers are blocked by collision, because the rapidly conducted potentials already have passed the distal site of stimulation, leaving a CMAP contributed by only the fast-conducting fibers. A plot of the amplitude of the CMAP (elicited by the proximal stimulus) against the interval between the two stimuli provides an estimate of the range of conduction of the fibers in the nerve. In the F-wave method, the range of latencies of F waves is measured with separate stimulation at proximal and distal sites. Comparison of the earliest and latest F-wave latencies at the two sites of stimulation and the distance between them provides a measure of conduction in the fast- and slow-conducting fibers.
With repetitive stimulation the area and amplitude of a CMAP are normally constant. In disorders of neuromuscular transmission and in some disorders of nerve and muscle, the area, amplitude, configuration, or latency of consecutive CMAPs may change with repetitive stimulation by either an increment or a decrement in the CMAP. A decrement is measured as a percentage change in the amplitude or area between two CMAPs recorded sequentially on stimulation at the same site. A decrease in amplitude from 10 to 9 mV is a decrement of 10 percent. A decrement at slow rates of stimulation (2 to 5 Hz) usually is caused by disease at the neuromuscular junction. Stimulation (or voluntary activation) at rapid rates (10 to 50 Hz) may result in decrements in normal people or in persons with disease of the neuromuscular junction, nerve, or muscle. Rapid rates of stimulation also may produce an increment in amplitude or area, which also is measured as a percentage change. An increase from 5 to 10 mV is an increase of 100 percent. Area and amplitude changes also may occur slowly after exercise in disorders such as periodic paralysis ( Fig. 13-3 ). Such changes are dependent on the rate of stimulation and are discussed in Chapter 17 .

When a peripheral nerve is stimulated, late waveforms may be elicited in addition to the direct M response ( Fig. 13-4 ). A nerve stimulus normally evokes action potentials that propagate proximally toward the anterior horn cell (antidromic), as well as peripherally (orthodromic) to activate the muscle directly. The antidromic potentials may be blocked by hyperpolarization at the anode, but if the cathode is placed proximally, all the motor axons can be activated antidromically. A small proportion of the anterior horn cells are activated antidromically in either the cell body or the axon hillock; these discharge another action potential orthodromically along the axon. Paired stimuli will block these waves because the second antidromic potential will collide with the recurrent orthodromic potential. These recurrent discharges produce a small muscle potential after a delay of 20 to 50 msec, depending on the distance from the site of stimulation to the spinal cord. These late responses are referred to as F waves ( Fig. 13-5 ) and were first described by Magladery and McDougal.


Individual F waves are the action potentials of a single or a few motor units. Although F waves represent only a small sampling of the axons in the nerve, and they have variable latencies as different axons are activated, they can provide an estimate of conduction in the central segments of motor fibers. F-wave latencies are assessed most readily by comparison of the measured latency with normal values at the same distance. F-wave latency values in normal subjects depend on the peripheral conduction velocity and the length to the limb. Estimates of normal values can be obtained readily by doubling the distance from the distal site of stimulation to spinal cord (sternal notch for arm and xiphoid for leg), dividing the result by the peripheral conduction velocity, and adding the distal latency. Values longer than the normal range of F estimates indicate proximal slowing relative to distal, as in a polyradiculopathy; those shorter than the F estimate indicate distal slowing relative to proximal, as in a peripheral neuropathy.
Individual F-wave latencies can be measured to obtain a statistical distribution with a mean, standard deviation, and range. These are time-consuming to collect, but they provide a more accurate measure of the range of velocities in the motor axons and an estimate of conduction in the slower-conducting fibers. Although samples of 20 F waves are needed to provide values of minimum and mean latency within 95 percent of the true value, 8 to 10 responses are sufficient to provide reliable values, and the procedure is tolerated readily by most patients. The F-wave amplitudes represent the summated potentials of the muscle fibers of one or more motor units and therefore have different amplitudes and configurations. The amplitude of a group of F waves provides an estimate of the relative amplitudes of the action potential of individual motor units. These also are described best by a combination of means, standard deviation, and maximal and minimal values. If there are only a small number of axons, the F waves of single axons may fire repeatedly to produce identical-appearing F waves from a single motor unit ( repeater F waves ) that are a sign of an underlying neurogenic process with collateral sprouting.
Other small electrical responses may occur after the M wave. For instance, if a few axons are conducting at a slower rate than the remainder, a small late potential may be seen after and time-locked to the CMAP; this is called an M-wave satellite . A second small response that may be confused with an F wave is an A wave . This results either from an axon that branches in the peripheral nerve; by spontaneous backfiring of an axon along its length; or by ephaptic activation of one axon by another, called direct and indirect double-discharges . Electrical stimulation distal to the site of branching produces an antidromic potential that becomes orthodromic at the branch point (see Fig. 13-4 ; Fig. 13-6 ). This potential, like the F wave, has a shorter latency as the site of stimulation moves proximally. The axon branch is typically a small, poorly myelinated, high-threshold axon, but a stimulus of sufficiently high intensity may activate the branch and block the A wave by collision. A third type of late response occurs in some diseases of peripheral nerve in which there is unusual irritability. In these diseases, the nerve and muscle discharge repetitively in response to a single stimulus. Each of these discharges must be distinguished carefully from F waves.

The CMAP is the summed response of the potentials of the individual motor units in the muscles activated by the stimulation. In normal people, the individual motor unit potentials are 20 to 200 μV in amplitude when recorded with surface electrodes. These are not recognized in standard nerve conduction studies. However, if a high amplification of the CMAP is combined with fine control of the stimulus intensity near threshold, the individual all-or-none firing potentials of single motor unit potentials can be isolated as step increments in the potential with increased stimulus ( Fig. 13-7 ). The number of motor units in a muscle can be estimated by measuring an average size of a single motor unit potential with these increments, then dividing that average into the size of the supramaximal CMAP. A number of automated methods have been developed to make this measurement. A mean of 320 (100 to 500) motor units is found in thenar and hypothenar muscles, and a mean of 150 (50 to 300) motor units is found in the EDB muscle. These measurements become less reliable with smaller motor units, as in myopathies, but become more reliable and reproducible with neurogenic atrophy. When the motor unit potentials become large, as in amyotrophic lateral sclerosis or after poliomyelitis, motor unit estimate measurements can be used to follow the course of the disorder quantitatively.

Nerve excitability is a more recent development in the testing of peripheral nerve. The method, which is discussed in Chapter 15 , is more complicated than standard nerve conduction studies. It measures a number of membrane characteristics related to threshold, including the effect of a test current on the nerve. Automated equipment has been developed for clinical testing. Although still early in its development, it has shown abnormalities in a number of clinical disorders.
Sensory nerve conduction studies
Stimulation and recording directly from a mixed peripheral nerve tests both motor and sensory axons. Evaluation of sensory axons alone requires stimulating and recording from a cutaneous nerve; recording from a cutaneous nerve while a mixed nerve is stimulated; recording from a mixed nerve while a cutaneous nerve is stimulated; or recording from the spinal column or cerebral hemispheres while a cutaneous or mixed nerve is stimulated. Sensory nerve action potentials (SNAPs) are of much lower amplitude than are CMAPs and may be obscured by other electrical activity and artifact. Averaging multiple responses sometimes is needed to provide reliable, measurable sensory potentials. Potentials that are recorded over the spinal column and scalp are called somatosensory evoked potentials (SEPs); these are discussed in Chapters 26 and 27 . SEPs recorded for the diagnosis of peripheral nerve disease are an extension of sensory nerve conduction studies. Sensory conduction in severe sensory neuropathies in which no SNAP is recordable can sometimes still be measured by recording the SEP.
SNAPs can be recorded readily from the ulnar, median, radial, lateral, and medial antebrachial, fibular, plantar, and sural nerves. SNAPs are also recorded preceding a CMAP at high amplification, if the muscle and overlying skin are innervated by the same nerve ( Fig. 13-8 ). Sensory recordings from the lateral antebrachial, saphenous, and lateral femoral cutaneous nerves are more difficult to obtain and are rarely of help in routine clinical studies.

Sensory conduction studies can add much to motor conduction studies (e.g., evidence of diffuse sensory fiber involvement, localized lesions involving a cutaneous nerve, or disorders that preferentially damage the sensory fibers in a mixed nerve). Because sensory nerve conduction studies are more sensitive than are motor conduction studies in detecting early or mild disorders, they are a necessary part of any electrophysiologic evaluation of peripheral neuromuscular disease.
Two other techniques, the blink reflex and the H reflex, assess sensory axons of the trigeminal nerve, median nerve, and sciatic nerve; these techniques are discussed in Chapters 18 and 19 . In each technique, sensory fibers are stimulated while a recording is made of a reflex motor response mediated by the central nervous system.
Stimulation
Because the speed of conduction in axons is the same orthodromically and antidromically, action potentials may be evoked in either direction for testing sensory conduction ( Fig. 13-9 ). Each has advantages and disadvantages that make it more appropriate for particular clinical situations. Stimulation of a cutaneous nerve at a distal site (e.g., the digital nerves in a digit) produces a small orthodromic nerve action potential that can be recorded over the proximal mixed nerve that it joins. Stimulation also can be applied over a mixed nerve to record a larger antidromic nerve action potential over the cutaneous nerve, but the latter may be associated with a motor response that is difficult to distinguish from the SNAP ( Fig. 13-10 ).


Stimulation parameters for sensory nerve conduction studies are similar to those for motor studies, and stimuli should also be supramaximal. The location and orientation of the stimulating electrodes are important for obtaining artifact-free recordings.
Recording
Recordings of compound SNAPs are technically more difficult to make than are those of CMAPs because of the smaller size of the potentials. Recording electrodes placed on the surface over a mixed or cutaneous nerve (particularly ring electrodes placed over the digits) are most convenient; however, needle recording electrodes placed near the nerve can enhance the amplitude of the response up to fivefold ( Fig. 13-11 ). Small components recorded with needle electrodes are generated by demyelinated or regenerated fibers that may be the only findings in some neuropathies. The nerve action potential is typically triphasic with a positive onset; it has latency proportional to the distance from the stimulating electrodes and an amplitude approximately proportional to the number of active axons, the synchrony of axonal firing, and the distance from the nerve to the recording electrodes. The distance of the electrode from the nerve can be estimated from the rise-time; nearby nerves will have rise-times of less than a millisecond.

The high amplification used for recording makes the stimulus artifact a more common problem. To reduce such artifact, greater attention is required for placement of ground electrodes, elimination of conducting bridges between the ground and the stimulating electrodes, isolation of the stimulating electrodes, and proper orientation of the stimulating and recording electrodes. Another common problem in recording sensory potentials is the appearance of background muscle activity, which is reduced most effectively by gentle manipulation of the limb and by providing patients with auditory feedback of their own muscle contractions. Sensory studies require particular attention to temperature because the superficial location of cutaneous nerves makes it more likely that mild slowing in conduction may be caused by low temperature.
Technically, the easiest and most reliable distal SNAP recordings for both the ulnar and the median nerves are obtained by orthodromic activation of axons. Radial and sural nerves, however, are tested more readily with an antidromic volley; recording is performed over a distal branch while the main trunk of the cutaneous nerve is stimulated. Simultaneous recording from more than one distal branch of a cutaneous nerve with antidromic stimulation may enhance sensitivity to localized nerve damage.
The placements of the active electrode and the reference electrode are of critical importance in recording SNAPs. The potential is optimal when the active electrode is immediately adjacent to the nerve and the reference electrode is placed as far away as possible. However, artifacts from intervening muscle or other tissue increase when the reference electrode is moved away. A compromise between optimal SNAP amplitude and an artifact-free signal must be reached. A reference electrode located 4 cm from the active electrode provides almost full representation of the SNAP with a minimum of noise. The reference electrode may be placed either laterally away from the nerve or longitudinally further along the course of the nerve. The latter placement leads to an inverted SNAP (near-field potential) being recorded from the reference electrode. At 4 cm, the inverted reference potential summates with the potential at the active electrode and increases the size of the signal; some distortion results if the active and reference electrodes are too close together.
However, in lateral placement, there is the more difficult problem of recording a far-field potential, which can distort the SNAP in an unpredictable fashion. The far-field potential may be recorded from the reference electrode before the action potential is recorded from the active electrode. The problem of recording far-field potentials from a laterally located reference electrode is less when recording is done with near-nerve needle electrodes because the far-field potential is relatively smaller than is the near-field potential, and it also causes less distortion. Needle electrode recordings also can provide a better representation of the components of the SNAP when the potential is broken up by dispersion. Such apparently dispersed SNAPs with multiple components recorded from either a needle or a surface electrode must be interpreted with caution because they are sometimes artifacts of the recording that are not reflected in either in vitro recordings from whole-nerve biopsy specimens or the distribution of axonal diameters in specimens from nerve biopsies. The multiple components may be artifacts either of stimulation of more than one nerve by an excessively large stimulus ( Fig. 13-12 ) or of recording a far-field potential ( Fig. 13-13 ).


Measurements
Latency, conduction velocity, area, and amplitude are measured for sensory conduction studies, as they are for motor conduction studies, but these are more difficult to measure because of configuration changes and small size. The SNAP is a moving wave recorded in a volume conductor and, therefore, typically has an initial positivity because of current flow ahead of the area of depolarization. The amplitude of the response measured from positive to negative peak provides the best estimate of the total number of fibers activated, although the distance between the recording electrode and the nerve heavily influences the amplitude. Area of the negative spike is a less satisfactory estimate because of the difficulty in defining measurement points.
The latency of the response is related directly to the rate of conduction and the distance between the stimulating and the recording electrodes. Comparisons of differences in latency and distance at different sites allow calculations of conduction velocity. Sensory compound nerve action potentials range in amplitude from a few microvolts to 200 μV. Velocities are generally faster than are motor conduction velocities. With distal stimulation, potentials are small when recorded at proximal sites and, at times, this precludes recording them directly without averaging. Larger potentials can be obtained with stimulation of the mixed nerve or by stimulation of unbranched digital nerves (e.g., the median or ulnar nerves in the palm). With palmar stimulation, orthodromic sensory potentials can be recorded at proximal sites without averaging ( Fig. 13-14 ).

The major problem in measuring the SNAP is its low amplitude, which makes the noise in the recording system a significant percentage of the total waveform. Therefore, the measurement of a single SNAP is unreliable, unless the noise level is very low or the amplitude is greater than 25 μV. Otherwise, averaging is needed. An automatic averager is of major value in measuring the SNAP. The averaged SNAP typically is a triphasic wave. The initial positivity is caused by the current from the potential as it approaches the active electrode, and the late positivity is the current flow of the potential moving away. These positive components are therefore of little diagnostic value. SNAPs have an initial negativity when recorded at the boundary of volume conductors of different sizes (e.g., the potential recorded from the digits).
Latencies of SNAPs are measured to the onset of the negativity, regardless of whether there is an initial positivity. If there is a positivity, the onset of the negativity is taken as the peak of the preceding positive phase. Latency to the onset reflects conduction in the fastest fibers and is used to calculate conduction velocity. Latencies to the negative peak may also be measured because they are defined more easily, especially in noisy recordings. Peak latencies will estimate conduction in the fibers with medium-range velocity. Peak latencies cannot be used to calculate conduction velocity from two points because the axons that produce the peak at the two sites differ as a result of dispersion of the potential. Latencies are measured most precisely with needle electrodes placed near the nerve, but surface measurements are also reliable if the electrode is placed properly to give a fast rise-time.
Conduction velocity in the fast-conducting sensory fibers is measured most accurately (as it is for motor conduction) by using the distance and latency between two points of stimulation. Unlike a motor latency, however, a sensory latency does not include the time for neuromuscular transmission, thereby allowing calculation of sensory conduction velocity by the distance and latency with stimulation at a single site. Although it is reliable, one-point velocity calculation still may include some slowing in nerve terminals. A lesion can be localized more precisely by an abrupt change in latency and in waveform of the antidromic sensory potential during “inching” of the stimulus in short increments along the nerve.
Measurements of conduction in the slower-conducting fibers of sensory nerves have been attempted with methods similar to those used in motor conduction studies. The collision method, in which paired stimuli are delivered at two sites along a nerve, gives estimates of conduction in slow sensory fibers. In Figure 13-15 , a full-size potential is obtained with intervals greater than 5 msec between stimuli separated by 14 cm. This indicates a minimal conduction velocity of 28 m/sec. All axons are blocked at intervals of less than 3.5 msec between stimuli for a maximal conduction velocity of 40 m/sec. These measurements can be technically difficult because of the need to average the potentials. A second method that has been used to estimate the conduction in small fibers uses a mathematical analysis of the configuration of the potential. This method has been shown to have some validity when compared with other methods, but it is fraught with unsolved technical problems, such as the distortion caused by the presence of far-field potentials recorded from the reference electrode.

The amplitude and area of the SNAP are important parameters that provide information about the number of axons and their sizes. They cannot be related directly to the number of axons, however, because the distance of the nerve from the electrode influences them. Surface electrodes can give estimates of the number of axons if they are placed near the nerve, as defined by the rise-time. Responses recorded with needle electrodes are more difficult to interpret because the amplitude, area, and configuration are so heavily dependent on the precise location of the electrode relative to the nerve; however, they can be used if they have fast rise-times. Responses recorded with needle electrodes also show turns, late components, and dispersion in the SNAP that are not seen with surface electrodes. Thus, needle-electrode and surface-electrode recordings of SNAP each have advantages and disadvantages.
The greater range of conduction velocities in sensory axons results in more cancellation of the negative phase of the SNAP by the initial and late positivity as the potential travels longer distances. Therefore, marked reductions in amplitude and area of SNAPs may occur with increased conduction distances, making such measurements less useful, except over distances of 10 cm or less. Decrement in amplitude and area with repetitive stimulation is also less useful because it occurs only with axonal disease and requires rapid rates of stimulation.
Other measures of sensory nerve conduction may be obtained by recording H-wave latency (see Chapter 18 ) or SEPs (see Chapters 26 and 27 ).
General principles of nerve conduction studies
Physiologic Variables
Normal values for latency, area, amplitude, and conduction velocity vary with age, height, temperature, and technique. Therefore, each laboratory should determine its own set of normal values. Median and ulnar nerves, and tibial and fibular nerves, have similar conduction velocities to each other and between the limbs for each nerve. Leg nerves conduct 7 to 10 m/sec slower than those in the arms, probably because of slower conduction in longer axons. Conduction in proximal nerve segments is somewhat faster than in distal segments.
Low temperatures result in slower conduction velocities and longer distal latencies; both nerve and muscle action potential amplitudes are higher with lower temperatures. Room temperatures above 70°F (20°C) minimize these changes, but use of a heating lamp or immersion of the hand or foot in water at 40°C before testing may be necessary if they feel cool to the touch. Continuous measurement of skin temperature (above 31°C in the hand and above 29°C in the leg) in the region of a nerve conduction study is necessary to ensure that responses are not altered by temperature.
Conduction velocities change markedly with age between the neonatal period and 6 years, with more gradual subsequent changes up to adulthood. Conduction begins to slow minimally after 40 years of age, but more so after the age of 60 years. Normal value determinations should be age corrected. Conduction velocity in the legs slows with height and should be corrected for heights over 78 inches (198 cm). Gender differences are caused primarily by differences in height.
Long- and Short-Segment Studies
Motor and sensory nerve conduction studies can be performed over different lengths of nerve. Usually, the length is selected for ease and reliability of testing (e.g., the elbow-to-wrist segment in the arm and the knee-to-ankle segment in the leg). However, both stimulation over short segments and stimulation over long segments have distinct advantages in certain clinical situations. A localized area of abnormality, either slowing or amplitude change, is most apparent if the testing isolates that segment from immediately proximal and distal segments. In longer segments, such local abnormalities may not be apparent because they are averaged with the normal segments. Short-segment testing is best accomplished by “inching” (i.e., stimulation at short intervals of 2 cm along a nerve).
In contrast, recording over long distances that summate abnormalities is the best way to recognize diffuse abnormalities, especially if they are mild. Longer segments also reduce the effect of small errors in distance or latency measurement. F-wave latencies assess the longest segments, and therefore are often more sensitive and accurate in identifying generalized slowing than standard peripheral conduction.
Distinguishing Proximal and Distal Abnormalities
Peripheral nerve disorders may produce slowing in distal segments (peripheral neuropathy), in proximal segments (polyradiculopathy), or in both segments. Distal abnormalities are identified readily with standard nerve conduction studies of limb nerves that involve stimulating and recording conduction velocity and distal latency from distal segments of the nerve. Identification of proximal abnormalities requires stimulating proximal nerves such as the musculocutaneous or facial nerves, or stimulating across plexus segments by percutaneous stimulation of spinal nerves combined with distal nerve stimulation. F-wave latency comparisons with F-wave estimates are most efficient for recognizing proximal slowing, as in a polyradiculopathy. Blink reflexes are also important for recognizing proximal slowing.
Various methods of calculation have been devised to obtain estimates of conduction velocities to identify the presence or absence of proximal slowing in combination with peripheral slowing. Comparison of F-wave latency with an estimated F-wave latency that is based on peripheral conduction and length of the nerve to the spinal cord can determine whether conduction slowing is equal proximally and distally, is greater distally than proximally, or is greater proximally than distally (see F-wave section, earlier).
Data Analysis
The analysis and interpretation of the numerical data obtained in nerve conduction studies depend on the reliability of the data and on the normal values with which they are compared. These are ignored too often, leading to erroneous conclusions about the presence or absence of peripheral nerve disease. Reliability is the responsibility of electromyographers, who must define the reproducibility of the data they obtain. This starts with careful attention to eliminating technical errors but then must be assessed formally. Repeating the nerve conduction studies on the same individual a number of times on different days can do this most readily. That variation then defines how to interpret patient data. For example, an ulnar/hypothenar CMAP that is 5 mV, when the lower limit of normal is 6 mV, cannot be considered abnormal unless the reproducibility is less than 1 mV. Amplitude measurements have the poorest reproducibility; distal latency, motor and sensory conduction velocities, and F-wave latency have increasing reproducibility.
A definition of normal values for an individual requires standard nerve conduction testing on a large population of normal subjects whose age, gender, height, and weight are recorded. Automated programs then can give percentiles and normal deviates based on a comparison of the findings in that patient with findings in normal subjects. A percentile analysis provides the percentage of matched normal subjects with that value rather than absolute normal values. In diffuse disorders (e.g., a peripheral neuropathy), the extent of abnormality may be different for different nerves tested. In those situations, assessing the progression of chronic peripheral neuropathies is enhanced by calculating composite scores of the motor or sensory amplitudes or latencies to provide a better picture of the overall status of the disease.
Technical Errors
Technical problems are a common source of error in motor and sensory nerve conduction studies. Any unexpected finding should be assumed to be caused by a technical error until proven otherwise. Spread of current because of excessive stimulation, small responses because of submaximal stimulation when the stimulator is not over the nerve or the nerve is deep, shock artifact, inadequate skin preparation, distance measurement errors, incorrect limb positioning, machine filter settings, inappropriate normal values, and incorrect location of recording electrodes must be watched for and eliminated.
The difficulty of measuring small responses or excessive background noise can lead to errors in measurement of action potentials. Averaging should be used for such signals. Small signals within the noise level of the system require averaging to improve the signal-to-noise ratio. Averaging sums a large series of responses that are time-locked to the stimulus. Random noise will be canceled out, whereas the consistent response becomes more apparent. Averaging is limited to eliminating background noise less than 50 times the signal size.
Risks
Nerve conduction studies are essentially risk-free in normal individuals when using equipment that is grounded properly. Nerve stimulation can be a risk if the current can reach the heart. It is thus relatively contraindicated in patients with catheters inserted directly into the heart. Limb stimulation is safe, but stimulation near the heart, such as phrenic nerve stimulation, should be avoided in patients with pacemakers, cardioverters, and defibrillators.
Anomalies of innervation
In standard nerve conduction studies, it is assumed that a patient’s nerves follow the normal anatomic patterns. However, as many as 20 percent of people may have anomalous patterns of innervation in the arm or leg; this can result in unusual findings on nerve conduction studies. If these variations are not recognized, they may be mistaken for disorders of peripheral nerves. Two anomalies that are of particular concern in nerve conduction studies are the presence of median–ulnar anastomosis in the forearm (Martin–Gruber anomaly) and a deep accessory branch of the superficial fibular (peroneal) nerve in the leg.
Median–Ulnar Anastomosis
Between 15 and 20 percent of normal people have anomalous axons that pass from the median to the ulnar nerve in the proximal third of the forearm, the so-called Martin–Gruber anastomosis ( Fig. 13-16 ). These axons leave either the main trunk of the median nerve or the anterior interosseous nerve and join the main trunk of the ulnar nerve. Axons leaving or joining a nerve between two sites of stimulation cause an unanticipated change in the size or shape of the CMAP ( Fig. 13-17 ). With ulnar nerve stimulation, the amplitude is lower at more proximal sites of stimulation, whereas with median nerve stimulation, the amplitude is higher at more proximal sites of stimulation. The axons that cross from the median nerve to the ulnar nerve may innervate any of several intrinsic hand muscles, but most commonly they innervate the first dorsal interosseous muscle. For standard nerve conduction studies, the most important sites of innervation are the thenar and hypothenar muscles ( Fig. 13-18 ). Therefore, this anomaly can be particularly confusing in cases of carpal tunnel syndrome, and it can mimic an ulnar neuropathy. The anomaly should be sought whenever the amplitude of the response to median nerve stimulation is higher with elbow than with wrist stimulation, when there is an initial positivity of the median motor response to stimulation at the elbow, or when the response to ulnar stimulation is lower in amplitude at the elbow than at the wrist. Sensory fibers cross in the anastomosis only rarely.


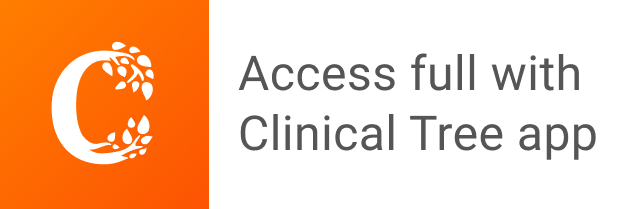