Nervous System Architecture in Vertebrates
Mario F. Wullimann
9.1 Introduction
The vertebrate brain has a distinct conservative bauplan that consists of a limited number of major brain parts and functional systems shared by all extant vertebrate species. Although some elements of this bauplan have been recognized for over a century, there were continued debates about possible additions of anterior brain elements, mostly in the context of a linear “scala naturae” view of brain evolution. Molecular genetic work corroborated many earlier ideas, and brought additional insights into an extended concept of a vertebrate brain bauplan. The current view is that vertebrate brain evolution has occurred by building brains in various lineages based on a fundamental bauplan rather than by sequential addition of new brain parts. During early development, this bauplan is represented by an array of histogenetic units ‐ proliferative zones arranged at the ventricular side of the neuroepithelial wall of the neural tube ‐ from which the adult structures arise by radial or other types of cellular migration and subsequent differentiation. Modern gene expression and functional studies have begun to unravel the complexity of the genetic machinery acting differentially in these histogenetic units. This work has helped to establish firmer conclusions regarding homologies of brain structures arising from particular histogenetic units, while at the same time revealing cases of homoplastic (convergent) evolution of brain characters in vertebrates. Most current debates involve the forebrain, and in particular the telencephalon. In this chapter, after describing the basic bauplan of the vertebrate brain, I will focus on the comparative neural architecture of the forebrain in major vertebrate taxa, in particular of the telencephalon, reviewing functional neuroanatomy, sensory inputs, and premotor/motor outputs, and discussing basal ganglia organization.
9.2 Natural Brain Units: Vesicles/Neuromeres and Longitudinal Columns
What are the morphological and functional units of the brain? In classical embryology the vertebrate brain is said to develop from the anterior portion of the neural tube, which passes from a two‐vesicle into a three‐vesicle stage (rhombencephalon, mesencephalon, prosencephalon) and then into a five‐vesicle stage whereby the prosencephalon or forebrain develops into telencephalon and diencephalon, and the rhombencephalon or hindbrain develops into metencephalon and myelencephalon (see Figure 9.1A and Butler & Hodos, 2005). These five brain parts are also recognized in adult vertebrates (see Figure 9.2). The rediscovery in the late 20th century of the equally old neuromeric paradigm established that the vertebrate rhombencephalon develops from seven to eight transitory neuromeres (called rhombomeres, Rh 1–8; plus possibly three more “cryptorhombomeres”; Marín, Aroca, & Puelles, 2008), and that the prosencephalon develops from at least three neuromeres (prosomeres, P1–P3; Puelles & Rubenstein, 1993, 2003), caudally, plus from a complex most anterior area called the secondary prosencephalon (see Figure 9.1B). Neuromeres are true transverse units of the central nervous system in the sense that they contain all dorsoventral parts of the neural tube at their anteroposterior axial location (see below). More specifically, rhombomeres are morphologically definable entities, with glial intersegmental boundaries and metameric sets of neurons and axonal branching (Holland & Hogan, 1988; Lumsden & Krumlauf, 1996; Wilkinson & Krumlauf, 1990). They furthermore represent compartments with clonal cell restriction (Fraser, Keynes, & Lumsden, 1990; Larsen, Zeltser, & Lumsden, 2001) and differential gene expression (Hunt & Krumlauf, 1992; Oxtoby & Jowett, 1993); neuromeres thus represent a useful topological framework to compare and interpret morphological observations across taxa. However, the vesicle and neuromeric (prosomeric) models are partially in conflict, both in regard to the number of transverse units and to the course of the longitudinal (anteroposterior) axis—and therefore also to the organization of longitudinal columns in the neural tube (Puelles & Rubenstein, 1993).

Figure 9.1 Vesicle and Neuromeric Models.
Schematics of embryonic mouse brain at 12.5 days in lateral views. (A) classical vesicle and (B) neuromeric (prosomeric) models. Red interrupted line: anteroposterior axis following hindbrain and forebrain flexures (see text).
Abbrevations: AP alar plate; BP basal plate; FMB forebrain–midbrain boundary; FP floor plate; MHB midbrain–hindbrain boundary; MTg midbrain tegmentum; P1—P6 prosomeres 1—6; Rh1–Rh8 rhombomeres 1—8; RP roof plate; sm somatomotor zone; ss somatosensory zone; vm visceromotor zone; vs viscerosensory zone; ZLI zona limitans intrathalamica.

Figure 9.2 Cladogram of Craniate Taxa and Illustrations of Representative Brains.
Note that three terms for nonmonophyletic groups are used in text: agnathans for myxinoids and petromyzontids, reptiles for amniotes except birds/mammals, and anamniotes for all craniates except amniotes.
Abbreviations: 1 SP first spinal nerve; a anterior cerebellar lobe; A accessory olfactory nerve; al anterior lateral line nerve; BO bulbus olfactorius; c central cerebellar lobe; Ce cerebellum; Di diencephalon; ds dorsal spinal nerve; EG eminentia granularis; H hypothalamus; Ha habenula; Hy hypophysis (pituitary); L lateral pallium (piriform cortex); MO medulla oblongata; MTg midbrain tegmentum; p posterior cerebellar lobe; PC pedunculus cerebri; pl posterior lateral line nerve; Spocc spino‐occipital nerve; Tel telencephalon; TeO tectum opticum; vs ventral spinal nerve. 0–XII as in Figure 9.3.
Three early embryonic vesicles reflect real transverse units. The midbrain–hindbrain boundary (MHB) is uniquely definable in molecular genetic terms as a transverse boundary region and an important signaling center (Brand et al., 1996; Marín & Puelles, 1994; Wurst & Bally‐Cuif, 2001). Similarly, the forebrain–midbrain boundary (FMB) has been described as exhibiting local gene expression (Scholpp & Brand, 2003) and acting in clonal cell lineage restriction (Larsen et al., 2001). However, the later division of the rhombencephalon into metencephalon and myelencephalon is mostly epiphenomenonal. In jawed vertebrates, a dorsally located cerebellum and, in both birds and mammals, a ventrally located palliocerebellar relay or pons, characterize the anterior hindbrain (metencephalon); these features distinguish the metencephalic portion of the medulla oblongata from the myelencephalic one. However, because the cerebellum is entirely within rhombomere 1 whereas the pons extends into rhombomere 3/4 (see Figure 9.1; Alonso et al., 2012; Aroca & Puelles, 2005), the metencephalon is not a true transverse brain unit. Similarly, the myelencephalon consists accordingly of unequal numbers of alar rhombomeric units (7) compared to basal plate units (4) and is, thus, also not a real transverse unit. Because a pons is only seen in birds and mammals, the adult rhombencephalon of anamniotes (all craniate taxa except amniotes) is sometimes divided into cerebellum and medulla oblongata (Figure 9.1A). A more severe problem relates to the prosencephalon. In the vesicle model, the telencephalon has been interpreted as lying in front of the diencephalon (Figure 9.1A). However, the existence of two neural tube flexures—one between midbrain and forebrain, the other (which is only pronounced in amniotes) between spinal cord and hindbrain—led to the recognition of the true longitudinal brain axis (Figure 9.1B; red interrupted line). An important consequence of this new axis is that the telencephalon is the dorsal part of the most anterior transverse neural tube unit and that the hypothalamus represents its ventral part, together forming the secondary prosencephalon (Figure 9.1B). The classical diencephalon of the five‐vesicle model (Figure 9.1A) is, therefore, not a true transverse unit. Moreover, the remainder of the diencephalon posterior to the hypothalamus forms three more transverse units (prosomeres, P1‐3): pretectum (P1), thalamus (P2), and prethalamus (P3, formerly ventral thalamus) (Figure 9.1B) (Puelles & Rubenstein, 1993, 2003). As mentioned above, clonal cell lineage restriction is present at the FMB (Larsen et al. 2001), as well as in the boundary zone of thalamus/prethalamus, i.e., within the zona limitans intrathalamica (ZLI) (Zeltser, Larsen, & Lumsden, 2001), another important transverse signalling region, which separates these two prosomeres as neuromeres (Figure 9.1B). Although clonal cell restriction has been demonstrated more stringently for rhombomeres than prosomeres, approximately 5% of cells also transgress rhombomeric boundaries (Birgbauer & Fraser, 1994). Furthermore, there is selective regulatory gene expression in P1 (Prox), P2 (Gbx2) and P3 (Dlx2) (Larsen et al., 2001). Moreover, early proliferation zones in the zebrafish brain also reveal these three prosomeres, while the secondary prosencephalon follows a more complex proliferation pattern (Wullimann & Mueller, 2004; Wullimann & Puelles, 1999).
What about longitudinal elements of the neural tube? The embryonic longitudinal columns in the spinal cord, that is the floor, basal, alar, and roof plates, represent the prototypical situation for the vertebrate central nervous system. They will develop into dorsal (roof plate) and ventral (floor plate) midline structures, and in particular—going from ventral to dorsal—into functional somatomotor and visceromotor (basal plate), as well as viscerosensory and somatosensory (alar plate) columns of the gray matter receiving sensory and giving rise to motor components of the spinal nerves, respectively (Figure 9.1B). These functional columns seemingly continue into the rhombencephalon and midbrain (Nieuwenhuys, 2011) because up to the midbrain tegmentum there are (basal‐plate‐derived) somatomotor elements (third nerve oculomotor nucleus) and alar‐plate‐derived sensory structures (optic tectum/superior colliculus and torus semicircularis/inferior colliculus). Anterior to this level, different paradigms have been used historically to explain the possible continuation of longitudinal zones (Puelles & Rubenstein, 1993; Shimamura, Martinez, Puelles, & Rubenstein, 1997). However, modern gene expression studies demonstrate how the domains of longitudinally expressed genes (such as sonic hedgehog and its downstream genes; Ericson et al. 1995; Shimamura, Martinez, Puelles, & Rubenstein, 1997) run in various longitudinal columns by following the neural tube flexures, thus demonstrating directly the new anteroposterior axis mentioned above. In the modern neuromeric model there is now consensus that all four embryonic longitudinal zones and their adult derivatives extend into the forebrain, and that the neural tube has its anterior tip in the area of the optic chiasm. Thus, the neuromeric model of Puelles and Rubenstein (1993, 2003) (Figure 9.1B) integrates approaches to divide the central nervous system into transverse and longitudinal zones and suggests a coherent definition of a vertebrate brain bauplan by proposing a matrix of transverse zones (neuromeres) along the anteroposterior axis which contain a segment of all longitudinal zones (an excellent historical account on these topics is found in Nieuwenhuys, 1998).
The outlined neuromeric model is of great heuristic value for a comparative discussion of the brains of vertebrates, which include jawless (agnathan) lampreys and jawed (gnathostome) vertebrates (see Figure 9.2). The latter include cartilaginous and ray‐finned fishes, actinistians (coelacanths), lungfishes, as well as tetrapod amphibians and amniotes (reptiles, birds, mammals). Craniates include additionally the myxinoids (slime eels, hagfishes). An example of a successful application of the neuromeric model is the development and adult location of primary motor nuclei in the vertebrate rhombencephalon which has resulted in improved understanding of developmental intergroup variability in the final adult arrangement of motor nuclei (Gilland & Baker, 2005). The trigeminal motor nucleus is formed and remains located in Rh2/3 in all vertebrates. The gnathostome facial motor nucleus is formed in Rh4 where it remains in adult anurans, while it migrates into Rh5 (and even Rh6) in all other gnathostome groups. This migration is under the control of Rh4 specific expression of the Hoxb1 gene (Lumsden, 2004). The motor nuclei of the oculomotor nerve (III) in the midbrain tegmentum and of the trochlear nerve (IV) in Rh1 are fixed landmarks in all vertebrate mid‐ and hindbrains. In contrast, the abducens motor nucleus neurons are located in Rh5/6 in lampreys, birds, and teleosts, but restricted to Rh5 in sharks, frogs, and mammals. Similar analyses exist for primary sensory and other rhombencephalic centers (Aroca & Puelles, 2005; Cambronero & Puelles, 2000; Marín & Puelles, 1995). This and other examples illustrate the power of the neuromeric model for the correct interpretation of both intergroup/interspecific diffences as well as commonalities in neural organization which otherwise would remain in the realm of contentious debate.
9.3 The Ancestral Bauplan of the Adult Craniate Brain
Despite great morphological differences between adult craniate brains (see Figure 9.2), the comparative phyletic method allows to recognize those characters which define its ancestral condition (bauplan or morphotype; Northcutt, 1985; Wicht & Northcutt, 1992). Analysis of adult characteristics, like the developmental ones reviewed above, suggests there was no “terminal addition” of brain regions to the anterior pole of the neuraxis (as envisaged by Ernst Haeckel’s recapitulation theory). Rather there was a basic brain bauplan at the outset of craniate evolution which was differentially modified in various taxa (as more generally proposed in pre‐Darwinian terms by Karl E. von Baer; see discussion in Butler & Hodos, 2005).
9.3.1 Hindbrain Cranial Nerves
The craniate hindbrain or rhombencephalon is ancestrally associated with the majority of cranial nerves (Figures 9.2 and 9.3 and Table 9.1) and related sense organs (reviews: agnathans: Braun, 1996; teleosts: Wullimann, 1998; cartilaginous fish: Hofmann, 1999). Craniate head development, including that of the cranial nerves and brain, is far more complex than that of the body trunk with respect to interactions of the three embryonic germ layers during neurulation (“theory of the new head”: Northcutt & Gans, 1983; see also Butler & Hodos, 2005). In particular, a third set of neuroectodermal structures are involved in cranial nerve development in addition to neural tube (somatomotor and preganglionic autonomic nerve components) and neural crest (sensory nerve components, postganglionic autonomic nerve components), both of which are involved in spinal nerve development as well. These third structures, called “placodes,” are embryonic epidermal thickenings of neurogenic tissues that give rise to most special sense head organs and—together with the head neural crest—to the cranial sensory ganglia and nerves.
The two most posterior of the 12 classically described human cranial nerves are present in craniates only among tetrapods: they are the hypoglossal nerve (XII, motor innervation of the tongue) and the accessory nerve (XI, motor innervation of two neck muscles). All vertebrates (including lampreys) possess the remaining ten cranial nerves and their primary motor and sensory centers: that is, the olfactory (I), optic (II), oculomotor (III), trochlear (IV), trigeminal (V), abducens (VI), facial (VII), otic (VIII), glossopharyngeal (IX), and vagal (X) nerves (see Table 9.1). The most anterior rhombencephalic cranial nerve (trochlear, IV), together with the abducens (VI) and the oculomotor nerve of the midbrain tegmentum (III), are somatomotor nerves and innervate the six extraocular eye muscles. The branchiomeric cranial nerves innervate one (V/VII/IX) or more (X) visceral arches (mandibular, hyoid, and gill arches or their derivatives; Butler & Hodos, 2005). The trigeminal nerve (V) carries most of the somatosensory information from the head surface (including proprioceptive signals) and oral cavity towards a principal and a spinal trigeminal nucleus. The facial (VII), glossopharyngeal (IX) and vagal nerves (X) all contain a gustatory component which innervates multicellular sensory organs called taste buds. These are located on the tongue in tetrapods and their primary sensory representation is in the medullary solitary tract nucleus. However, taste buds may be located outside of the tongue and oral cavity in fishes (such as goldfish or catfish) where they are always innervated by the facial nerve, whereas the oral cavity is subserved by the glossopharyngeal and vagal nerves. Depending on taste bud density, teleosts have more or less enlarged associated medullary primary sensory facial or vagal centers (FLo/VLo in Figure 9.3A). In gnathostomes, the branchiomeric cranial nerves always have a somatomotor component subserving the jaw musculature (V), the musculature of the hyoid arch (or its derivatives including the facial muscles of mammals, VII), and the gill musculature (or its derivatives, IX/X). Although the situation is basically similar in myxinoids and lampreys, their motor innervation of the trigeminal nerve is not to jaw muscles, but to specialized musculature associated with their convergently evolved feeding apparatus (Kuratani & Ota, 2008); an additional peculiarity unique to myxinoids is that they have (apparently independently evolved) skin taste receptor organs innervated by the trigeminal and spinal nerves (Braun, 1996). Branchiomeric cranial nerves display a viscerosensory and visceromotor (parasympathetic) component related to the innervation of head glands and visceral organs. The otic or vestibulocochlear nerve (VIII) innervates the mechanosensory inner ear (labyrinth) hair cells which subserve the vestibular senses (gravity, torsion); in all gnathostomes it additionally subserves at least a rudimentary sense of hearing (likely without directional hearing, except in amniotes). Details of mammalian cranial nerve components and their innervation sites are summarized in Table 9.1.
Table 9.1 Cranial Nerves in Mammals.
0 N. terminalis | olfactory epithelium VM/SS (?) |
I N. olfactorius | olfactory epithelium SS |
II N. opticus | retina SS |
III N. oculomotorius | ciliary muscle/pupillary sphincter VM 4 extraocular eye muscles: M obliquus inferior, M rectus superior/inferior/medialis SM |
IV N. trochlearis | 1 extraocular eye muscle: M obliquus superior SM |
V N. trigeminus | skin somatosenses: Face, oral/nasal cavity, tongue, teeth, plus proprioception SS jaw muscles and M tensor tympani SM |
VI N. abducens | 1 extraocular eye muscle: M rectus lateralis SM |
VII N. facialis | outer ear/auditory duct/tympanum (SS) tongue (taste buds) VS sublingual‐/submandibular gland VM facial musculature, M stapedius SM |
VIII N. octavus | inner ear (vestibular senses/hearing) SS |
IX N. glossopharyngeus | tongue, pharynx, middle ear SS tongue (taste buds) VS parotis gland VM pharynx SM |
X N. vagus | pharynx/larynx, outer ear/auditory duct, tympanum, meninges (dura mater), epiglottis SS inner organs, epiglottis (taste buds) VS inner organs VM pharynx/larynx SM |
XI N. accessorius | 2 neck muscles: M sternocleidomastoideus, M trapezius SM |
XII N. hypoglossus | tongue musculature SM |
SS, somatosensory (incl. special senses such as vision, olfaction, hearing, plus proprioception), VS, viscerosensory (incl. gustation); VM, visceromotor (parasympathicus); SM, somatomotor. Note that striated muscles derived from visceral arch musculature (the jaw, hyomandibular and gill arches) are considered somatomotor.

Figure 9.3 Classical Brain Parts and Differences Regarding Presence of Cranial Nerves.
Bodian‐Nissl stained sagittal adult zebrafish brain section (A) and schema of sagittal adult rat brain section (B) exemplifying classical brain parts and differences regarding presence of cranial nerves between anamniotes (zebrafish) and amniotes (rat).
Abbreviations: 0 nervus terminalis; I nervus opticus; II nervus olfactorius; III nervus oculomotorius; IV nervus trochlearis; V nervus trigeminus; VI nervus abducens; VII nervus facialis; VIII nervus octavus (or vestibulocochlearis); IX nervus glossopharyngeus; X nervus vagus; XI nervus accessorius; XII nervus hypoglossus; ac anterior commissure; BO bulbus olfactorius; CC crista cerebellaris; CCe corpus cerebelli; Corp call corpus callosum; DT dorsal thalamus; FLo facial lobe; Inf Coll inferior colliculus; H hypothalamus; Ha habenula; LL lateral line nerves; on optic nerve; MO medulla oblongata; P pallium; Po preoptic region; Pin pineal; poc postoptic commissure; PT posterior tuberculum; T midbrain tegmentum; S subpallium; SC spinal cord; Sup Coll superior colliculus; TeO tectum opticum; Va valvula cerebelli; VLo vagal lobe; VT ventral thalamus (prethalamus).
The ancestral vertebrate condition is characterized by some additional cranial nerves. The comparative and embryological study of placodes, their developmental fate, and the adult configuration of cranial nerves and sense organs resulted in a new understanding of the vertebrate head and its evolutionary history, due mostly to the work of Glenn Northcutt and coworkers (review in Northcutt & Bemis, 1993). The so‐called lateral line nerves (up to six in gnathostome fishes, Northcutt in Coombs, Görner, & Münz, 1989) innervate multicellular mechanosensory neuromasts (containing hair cells) and electroreceptors on the body surface (Bullock, Bodznick, & Northcutt, 1983). Similar to extraoral taste buds, lateral line organs are always innervated by their cranial nerves and never by spinal nerves. Thus, lateral line nerves are not part of the branchiomeric nerves, as was erroneously assumed in earlier decades. In fact, lateral line nerves fulfill all criteria for independent cranial nerves, as they have a distinct origin in specific placodes from which their peripheral ganglia and sensory organs develop. Also, distinct central nervous primary sensory nuclei are associated with them (Coombs et al., 1989; Wullimann & Grothe, 2013). Lateral line sensory organs and nerves are present together with an inner ear and octaval nerve in all vertebrates and appear, thus, to be equally old (Northcutt, 1986). Therefore, the inner ear did not evolve by internalization of a part of the superficial lateral line organs, as envisioned previously by the octavolateralis/acousticolateralis hypothesis. Also the concept of a medullary “octavolateral region” with overlapping acoustic and lateral line primary sensory nerve input is likewise clearly flawed. The ancestral vertebrate situation is, apparently, that separate primary sensory nuclei are present for electrosensory, mechanosensory, and auditory hair‐cell‐related modalities (McCormick, 1992). In sum, the medulla oblongata, together with the spinal cord, links the central nervous system, through the peripheral nervous system (i.e., all sensory and motor nerves described above) to most organ systems of the vertebrate body, enabling it to coordinate functions and enact behavior.
9.3.2 Cerebellum
The first rhomobomere of the gnathostome rhombencephalon includes a large, unique dorsal brain part: the cerebellum. It has a three‐layered cortex (deep granular, intermediate ganglionic [exhibiting Purkinje cells], and superficial molecular layers) that contains comparable cell types with a similar modular microcircuitry across all jawed vertebrates. The cerebellar afferent mossy fiber inputs (from vestibular nuclei, spinal cord, somatosensory lateral reticular/external cuneate, and accessory optic system nuclei) and efferent connections (to thalamus, nucleus ruber, vestibular nuclei, reticular formation) bear great resemblance among gnathostomes. Additionally, in mammals and birds, a ventrally located pons exists which is a relay center to the cerebellum for input from the mammalian cortex or its homologous area in birds. Furthermore, climbing fiber input from the inferior olive is present in all gnathostome vertebrates. This suggests that a cerebellum is ancestral for gnathostomes with similar functions in motor learning and coordination/execution. However, a palliopontine input and likely also a cerebellothalamopallial loop seems derived for birds and mammals. Interestingly, the cerebellum and its input nuclei share a common ontogenetic origin. The rhombencephalon has a dorsal (rhombic) groove, which is covered by the strongly vascularized neuroepithelial chorioid plexus that generates the cerebrospinal fluid. In gnathostome embryos, the rim of this groove is called the rhombic lip, representing the most dorsal area of the rhombencephalic alar plate. It gives rise, through extensive cellular migration, to all cerebellar projecting systems mentioned, plus the cochlear nuclei, and to the cerebellum itself (review: Wullimann et al., 2011).
9.3.3 Midbrain
The craniate midbrain or mesencephalon displays ancestrally a dorsal (alar plate) region, the tectum mesencephali, which is divided into an optic tectum (visual/multisensory; Figure 9.2) and a torus semicircularis (auditory/lateral line). In mammals, these paired structures are named superior and inferior colliculi (Figure 9.3B), with the former lying anterior to the latter (hence, corpora quadrigemina). However, in the remaining vertebrates, the optic tectum often expands in a dome‐like fashion over the torus semicircularis which thus comes to lie posteroventrally to the optic tectum (particularly in birds and teleosts). Although the optic nerve (II) is primarily associated with the diencephalon (see §9.3.4), in most vertebrates its major projection is to the superficial layers of the optic tectum/superior colliculus. The cytoarchitectonic and modular organization of the craniate optic tectum, which is a multilayered cortex, its segregated multimodal input, and the topographical representation of this input (in particular the visual one, plus additional sensory inputs) and output to the reticular formation provide very likely an ancestral neuronal machinery apparently exquisitely designed for integrative orientation tasks, such as object identification and location, and coordinated motor control (details for various taxa: see Nieuwenhuys, ten Donkelaar, & Nicholson, 1998; cartilaginous fishes: Hofmann, 1999; Northcutt, 1978; Smeets, Nieuwenhuys, & Roberts, 1983; ray‐finned fishes: Meek & Nieuwenhuys, 1998; Wullimann, 1998). The vertebrate torus semicircularis (including that of lampreys, González, Yáñez, & Anadón, 1999) is recipient to lateral line and/or auditory input via the lateral lemniscus from the respective primary sensory centers in the medulla. Even myxinoids have an ill‐defined posterior tectal area receiving bulbar lemniscal input (Wicht & Nieuwenhuys, 1998). The vertebrate ventral (basal plate) midbrain includes the midbrain tegmentum where motor structures are located, such as the oculomotor nerve (III) and its motor nucleus (Figure 9.2). The oculomotor nerve also includes a parasympathetic component (the Edinger–Westphal or accessory oculomotor nucleus, which controls pupillary light reflex). Other important basal midbrain centers in amniotes include the nucleus ruber, periaqueductal gray and dopaminergic ascending systems, that is, the substantia nigra/ventral tegmental area (Puelles, 2007) (see §9.4.2 for situation in anamniotes).
9.3.4 Forebrain
The classic concept of the “diencephalon” or “between‐brain” envisaged epithalamus (pineal, habenula), dorsal thalamus (with pretectum), ventral thalamus, and hypothalamus as a dorsoventral series of divisions within the embryonic diencephalic vesicle. In contrast, the neuromeric model (Puelles & Rubenstein, 1993, 2003) established that pretectum, dorsal thalamus (including epithalamus), and ventral thalamus (prethalamus) represent a series of transverse neural tube units arranged from posterior to anterior (prosomeres; see §9.2) with the hypothalamus (basal plate) and telencephalon (alar plate; including preoptic region) lying further rostrally. The posterior tuberculum of fishes develops from the basal plate portions of prosomeres 2 and 3, and the region of the nucleus of the medial longitudinal fascicle from the basal plate of prosomere 1 (Vernier & Wullimann, 2009). Both thalamus/prethalamus and hypothalamus are important integration/control centers present in all craniates. The hypothalamus is the integration center for autonomic‐visceral functions (body homeostasis), including the hormonal regulation of bodily functions via the pituitary. The thalamus is in receipt of sensory information from various brain regions, directly via the optic nerve from the retina, as well as through ascending sensory pathways from the midbrain and hindbrain. The functional interrelationship of thalamus and telencephalon will be discussed for each major taxon below. The optic nerve (II) enters the brain between preoptic region and hypothalamus. However, this nerve is in fact a brain tract, since the retina develops from the central rather than the peripheral nervous system. Retinal ganglion cell axons are, nonetheless, traditionally called the optic nerve up to the optic chiasm, and from then to the brain they form the optic tract.
The telencephalon (endbrain) of all craniates has a pallium (all parts of the mammalian cortex, pallial amygdala, and olfactory bulb) and a subpallium (septum and basal ganglia). The craniate amygdala comprises both pallial and subpallial telencephalic regions. The pallium includes multisensory integration centers, particularly pronounced in gnathostomes (details in §9.4 below). The craniate olfactory nerve (I) consists of axons of sensory olfactory epithelial cells (so‐called primary sensory cells unlike any other craniate nerve) which project to the olfactory bulb. All other sensory craniate (spinal and cranial) nerves have peripheral ganglia containing pseudounipolar neurons with a dendrite into their sensory organ and a centrally projecting axon. In tetrapods, the olfactory system is divided into a main olfactory epithelium/nerve/bulb and a vomeronasal or accessory epithelium/nerve/bulb; a similar functional subdivision may also exist, at the olfactory receptor cell level within an undivided olfactory epithelium, in ray‐finned fishes and, probably, cartilaginous fishes (Eisthen, 2004). A late‐discovered cranial nerve, the terminal nerve (0), is also associated with the telencephalon (Kawai, Oka, & Eisthen, 2009; Wirsig‐Wiechmann, Wiechmann, & Eisthen, 2002). In contrast to the olfactory nerve, the terminal nerve has ganglion cells (deriving from the cranial neural crest and/or olfactory placode) which have a neurite directed peripherally, into the olfactory epithelium/bulb, and another directed centrally (Schlosser, 2006; von Bartheld, 2004; Whitlock, 2004). These ganglion cells are heterogenous within vertebrate species with respect to location (near olfactory epithelium/bulb/within telencephalon) and to transmitters (gonadotropin‐releasing hormone, acetylcholine, glutamate; Edwards, Greig, Sakata, & Elkin, 2007; Kawai et al., 2009; von Bartheld, 2004). The function of the terminal nerve likely involves modulatory/parasympathetic innervation of the olfactory epithelium/bulb (Fujita, Sorensen, Stacey, & Hara, 1991), not pheromone detection (Demski & Northcutt, 1983).
9.3.5 Descending Premotor Systems
Finally, similar descending premotor systems in craniates are in control of the primary motor nuclei of the midbrain, hindbrain, and spinal cord which, altogether, are the sole neurons to directly release motor behaviors. At their simplest, motor behaviors are mediated by central pattern generators: small locally acting neuronal networks in spinal cord or medulla oblongata which organize reflexes (withdraw, cough, sneeze, swallow) or rhythmic movements (walk, chew, breathe; Grillner in Squire et al., 2008). Posture and eye movements involve already larger brainstem–spinal cord networks (e.g., reticulospinal, vestibulospinal, rubrospinal, tecto‐reticular); these locomotor patterns may be coordinated with one another and adapted to their environmental conditions, even without forebrain control (as evidenced by experiments with decerebrated mammals). However, brainstem premotor systems may be influenced by forebrain centers for more complex adaptive, goal‐directed behaviors. For example, hypothalamic centers guide eating, drinking, and aggression‐related behaviors (observed even in mammals without cortex; Grillner in Squire et al., 2008). Moreover, in amniotes, telencephalic (pallial/cortical) control over lower level behaviors is possible (from suppression of breathing to skillful learning‐dependent movement). Both cerebellum (in all gnathostomes) and basal ganglia (in all vertebrates) play complementary roles in these motor processes. Although long palliospinal and palliopontine pathways originated (independently) exclusively in mammals (pyramidal tract) and birds, all craniates possess various multisynaptic descending control systems. These travel from forebrain, via midbrain and hindbrain, to cranial and spinal nerve motor neurons (those involving the basal ganglia will be detailed in §9.4.1.3). As in amniotes, in cartilaginous (Smeets et al., 1983) and ray finned‐fishes (Wullimann, 1998), descending projections to the spinal cord arise from all parts of the reticular formation, the inferior (serotoninergic) raphe, vestibular and sensory trigeminal nuclei, and from the nucleus ruber. An important descending system is present in the nucleus of the medial longitudinal fascicle, an ancestral craniate premotor nucleus located in the basal part of pretectal prosomere 1, which projects to the medulla oblongata and the spinal cord and plays a crucial role in the stereotyped escape reflex (startle response). In mammals, the corresponding interstitial nucleus of the medial longitudinal fascicle plus the interstitial nucleus of Cajal are involved in head and eye movement control (Nieuwenhuys et al., 2008). Also myxinoids and lampreys have descending connections to the spinal cord from all parts of the reticular formation, and from vestibular and sensory trigeminal nuclei (Ronan, 1989). However, both agnathan taxa lack a nucleus ruber, likely because of an absence of pectoral and pelvic fins, the homologs of tetrapod limbs. In all craniates, the optic tectum (superior colliculus) and, in gnathostomes, the cerebellum act on premotor centers, in particular on the reticular formation.
9.3.6 The Agnathan Situation
Most of the above‐discussed vertebrate characteristics are also found in myxinoids, and are thus ancestral to all craniates. However, myxinoids lack a cerebellum, and even lampreys lack both Pax6 expression (required for gnathostome cerebellar development; Murakami, Uchida, Rijli, & Kuratani, 2005; Wullimann et al., 2011) and Purkinje cells (Lannoo & Hawkes, 1997) in the anterior rhombencephalic region (upper rhombic lip) where a small cerebellum was once suspected. However, recent gene expression studies suggest that a lower rhombic lip exists in agnathans (Sugahara et al., 2016). Another difference is that all jawed vertebrates have three semicircular canals in the inner ear labyrinth, whereas myxinoids have one and lampreys two. This may be directly related to the absence of any Otx gene expression in the otic cyst of agnathans, an interpretation supported by observations of Otx1 mutant mice, which lack the horizontal canal (Mazan, Jaillard, Baratte, & Janvier, 2000; Germot et al., 2001). Thus, since both agnathan groups lack many rhombic‐lip‐derived structures, including a cerebellum, these can be surmised to represent evolutionary novelties of gnathostomes. Moreover, myxinoids—but not lampreys—lack extraocular eye muscles, as well as the associated cranial nerves (III/IV/VI) and their motor nuclei. Finally, myxinoids lack both the terminal nerve and the electrosensory (but not the mechanosensory) component of the lateral line nerves (Braun, 1996; Wicht, 1996; Wicht & Northcutt, 1998). The absence of these neural characters likely represents the ancestral situation of craniates, rather than secondary losses, and highlights the position of myxinoids as the sister group to the vertebrates.
9.4 Comparative Brain Architecture in Craniates
Because the organization of the central nervous system is better known in amniotes than anamniotes, the following discussion will start with a comparison of mammals and birds/reptiles before going into various anamniote taxa.
9.4.1 Comparative Brain Architecture in Amniotes
Based on the shared bauplan of the craniate brain outlined above, which shows essential similarities in primary sensory input (spinal and cranial nerves/primary central nervous projection areas) and in final premotor output to motor nuclei of the brainstem (hindbrain and midbrain), the adult organization of the forebrain shall now be described with emphasis on two focal topics: first, the sensory pathways to telencephalon; second, the motor networks of the basal ganglia.
9.4.1.1 Correlation of brain size and complex cognitive behavior in birds and mammals.
Within craniates, birds and mammals have the largest brains relative to body size (discussed in Wullimann & Vernier, 2009b). Moreover, similarly sized avian and mammalian species share equally large brains. Even on superficial inspection, some macroscopical correspondences are apparent (e.g. compare duck and horse brains in Figure 9.2). A notable exception is that the large optic tectum of birds is displaced laterally relative to the mammalian homologue, the superior colliculus. Both mammal and bird brains have a large cerebellum and telencephalon (Northcutt, 2011), the pallium of which overgrows not only the rest of the prosencephalon but also most of the mesencephalon (see Figure 9.2).
In mammals, the telencephalic ventricle separates the isocortex (dorsal pallium) from the basally lying subpallium (which includes the basal ganglia, i.e., striatum and pallidum, plus septum; Figure 9.4A, B). In birds, massive telencephalic brain parts lie subjacent to the dorsal pallium (hyperpallium, formerly called the Wulst) and displace the telencephalic ventricle medially (Figure 9.4D). These neural masses, mesopallium and nidopallium (formerly called the dorsal ventricular ridge or DVR, as are their reptilian homologues), are basally bordered by subpallial structures, the basal ganglia (striatum/pallidum). Up to the mid 20th century, the DVR had been interpreted as an extended basal ganglionic region. This was in line with the then widely accepted view that birds display mostly hard‐wired, stereotyped, instinctive behaviors, in contrast to the plastic, learned, and cognitive behaviors of mammals. Because the mammalian basal ganglia were already then known to be involved in the initiation/selection and execution of motor activity (Mink, in Squire et al., 2008), the contemporary historical interpretation of the avian DVR was that birds need larger basal ganglia for the production of complicated, but inflexible, motor acts than do mammals, who instead have a large isocortex dedicated to flexible modification of learned behavior. This view of the DVR turned out to be neurobiologically flawed (Jarvis et al., 2005; Reiner et al., 2004); moreover, stunning reports now demonstrate the learning capabilities and cognitive capacity of birds such as ravens and parrots (Emery & Clayton, 2004). The functional neuroanatomical and developmental findings which parallel this modern view of bird brain and behavior will be discussed below, using recently updated terminology for bird brain regions (Reiner et al., 2004).

Figure 9.4 Transverse Nissl‐Stained Sections through Left Telencephalic Hemispheres of Mouse (Mus musculus, A: anterior, B: posterior), Tuatara (Sphenodon punctatus, C), Pigeon (Columba livia, D), Fire‐Bellied Toad (Bombina orientalis, E), and Zebrafish (Danio rerio, F). Schema shows partial eversion hypothesis (G). Major pallial and subpallial divisions are present in all gnathostome taxa. Arrows in D point to cell‐free laminae at boundaries between avian telencephalic divisions. See acknowledgments for origin of sections.
Abbreviations: ac anterior commissure; CP caudate‐putamen; Dc central zone of dorsal telencephalic area; Dl lateral zone of dorsal telencephalic area; Dm medial zone of dorsal telencephalic area; Dp posterior zone of dorsal telencephalic area; DP dorsal pallium; DVR dorsal ventricular ridge; L lateral amygdala; LP lateral pallium; MP medial pallium; NA nucleus accumbens; PA pallial amygdala (note fine white dots indicating parts of lateral pallial origin); Se Septum; SPA subpallial amygdala; Str Striatum; Vc central nucleus of ventral telencephalic area; Vd dorsal nucleus of ventral telencephalic area; Ve telencephalic (lateral) ventricle; Vl lateral nucleus of ventral telencephalic area; VP ventral pallium; Vs supracommissural nucleus of ventral telencephalic area; VT ventral thalamus (prethalamus); Vv ventral nucleus of ventral telencephalic area.
9.4.1.2 Ascending sensory pathways in birds and mammals.
Mammals have two major visual pathways to isocortex originating from retinal ganglion cells (Figure 9.5A). The dominating thalamofugal pathway is associated with detailed perception of visual clues and includes parallel processing streams for color/shape and movement running via the dorsal thalamus (lateral geniculate nucleus) to primary visual cortex (Brodmann area 17). The tectofugal pathway runs via the superior colliculus to the thalamus (pulvinar/lateral posterior nucleus) and from there to secondary visual cortex (areas 18/19). In mammals, this second pathway is associated with unconscious visual processing (blindsight) and is involved in the control of eye movements during object localization (Nieuwenhuys et al., 2008). Birds also have a thalamofugal pathway running to the hyperpallium (Wulst) via the dorsal thalamus (dorsolateral thalamic nucleus), but the dominant avian visual pathway is tectofugal (Engelage & Bischof, 1993; Remy & Güntürkün, 1991; Shimizu & Bowers, 1999), which courses through optic tectum and dorsal thalamus (nucleus rotundus) en route to the entopallium of the DVR (see Figure 9.5B). In lateral‐eyed birds, such as pigeons, the tectofugal pathway is the major perceptual processing stream for pattern recognition, innervating separate areas in the nucleus rotundus related to color, size, brightness, and movement perception (Bischof & Watanabe, 1997; Engelage & Bischof, 1993; Wang, Jiang, & Frost, 1993; Watanabe et al., 2008). Furthermore, in zebra finches, entopallial lesions lead to disruption of pattern recognition, while lesions of visual hyperpallium (Wulst) do not (though the latter do result in spatial discrimination deficits) (Watanabe et al., 2011).

Figure 9.5 Schematics of Sagittal Sections of (A) Rat and (B) Pigeon Brains Showing Ascending Visual Pathways (Rat after Nieuwenhuys et al. 2008, pigeon after Remy & Güntürkün, 1991; Engelage & Bischof, 1993; Shimizu & Bowers, 1999).
Abbreviations: DLT dorsolateral thalamic nuclei/principal optic nuclear complex; E entopallium (old name: ectostriatum); InfColl inferior colliculus; LGN lateral geniculate nucleus; LPN lateral posterior nucleus; NR nucleus rotundus; Olf Bulb olfactory bulb; TecOpt tectum opticum.
The somatosensory systems in birds and mammals also display comparable ascending pathways from primary sensory nuclei of spinal cord/medulla oblongata through thalamus to dorsal pallium (avian hyperpallium (Wulst)/mammalian primary somatosensory cortex) and, in birds, additionally to the DVR (Necker, 1989; Nieuwenhuys et al., 2008; Schneider & Necker, 1989; Wild, 1985, 1987, 1989, 1994; Wild, Arends, & Zeigler, 1984, 1985; Wild & Zeigler, 1996). In the mammalian thalamus, the dorsal column‐medial lemniscal system (label 2 in Figure 9.6
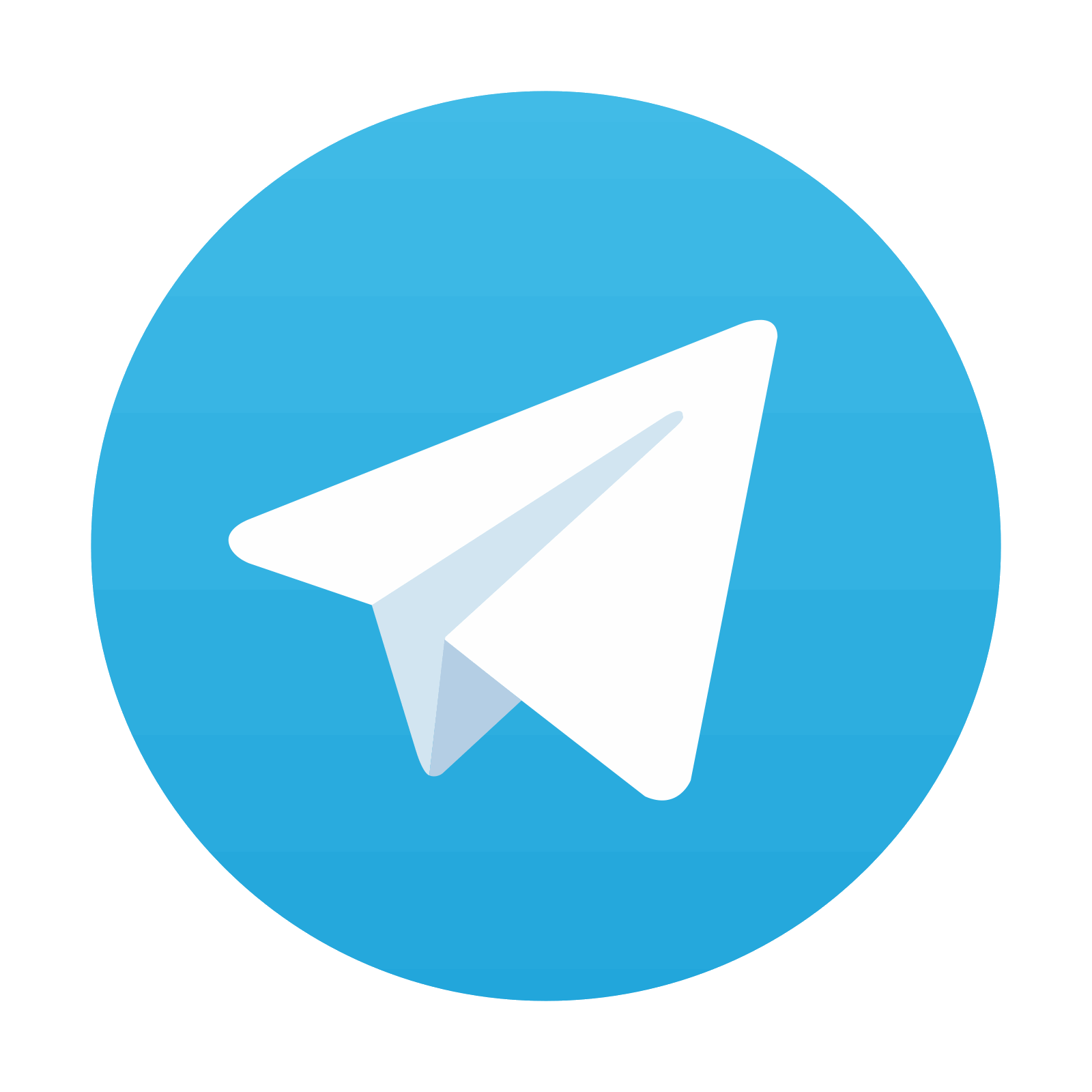
Stay updated, free articles. Join our Telegram channel
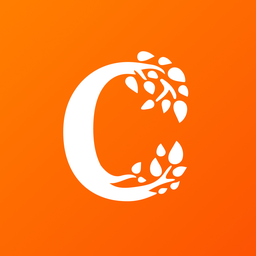
Full access? Get Clinical Tree
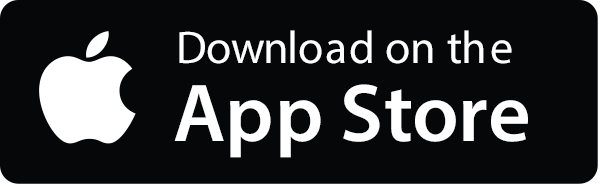
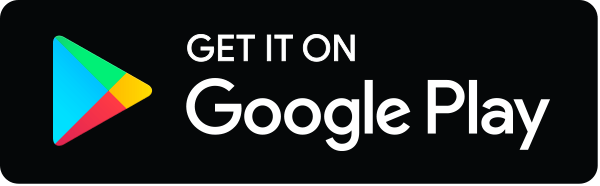