Chapter 33A Neuroimaging
Structural Imaging: Magnetic Resonance Imaging, Computed Tomography
Computed Tomography
By changing the settings of the process of transforming the x-ray attenuation values to shades on the grayscale, it is possible to select which tissues to preferentially display in the image. This is referred to as windowing. Utilizing a bone window, for instance, is very useful for evaluating fractures in cases of craniofacial trauma (Fig. 33A.1).
More than 20 years ago, a fast-imaging technique called spiral (or helical) CT scanning was introduced to clinical practice. With this technique, the x-ray tube in the gantry rotates continuously, but data acquisition is combined with continuous movement of the patient through the gantry. The circular rotating path of the x-rays, combined with the linear movement of the imaged body, results in a spiral or helix-shaped x-ray path, hence the name. These scanners can acquire data rapidly, and a large volume can be scanned in 20 to 60 seconds. This technique offers several advantages, including more rapid image acquisition. During the short scan time, patients can usually hold their breath, which reduces/minimizes motion artifacts. Timing of contrast bolus administration can be optimized, and less contrast material is sufficient. The short scan time, optimal contrast bolus timing, and better image quality are very useful in CT angiography, where cervical and intracranial blood vessels are visualized. These images can also be reformatted as 3D views of the vasculature, which are often displayed in color and can be depicted along with reformatted bone or other tissues in the region of interest (Fig. 33A.2).
Magnetic Resonance Imaging
Basic Principles
In clinical practice, MRI uses the magnetic characteristics inherent to the protons of hydrogen nuclei in the tissue, mostly in the form of water but to a significant extent in fat as well. The protons spin about their own axes, which creates a magnetic dipole moment for each proton (Fig. 33A.3). In the absence of an external magnetic field, the axes of these dipoles are arranged randomly, and therefore, the vectors depicting the dipole moments cancel each other out, resulting in a zero net magnetization vector and a zero net magnetic field for the tissue.
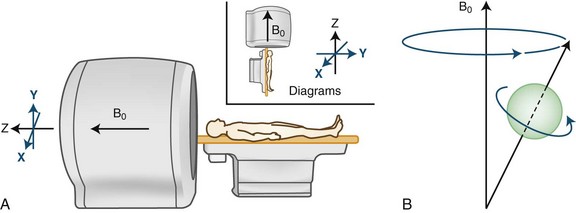
(A from Higgins, D., 2010. ReviseMRI. http://www.revisemri.com/questions/basicphysics/precession; B Reprinted with permission from Hashemi, R.H., Bradley, W.G., Lasanti, C.J., 2004. MRI—The Basics. 2nd ed. Lippincott Williams & Wilkins.)
This situation changes when the body is placed in the strong magnetic field of a scanner (see Fig. 33A.3, A). The magnetic field is generated by an electric current circulating in wire coils that surround the open bore of the scanner. Most MRI scanners used in clinical practice are superconducting magnets. Here the electrical coils are housed at near–absolute zero temperature, minimizing their resistance and allowing for the strong currents needed to generate the magnetic field without undue heating. The low temperature is achieved by cryogens (liquid nitrogen or helium). Most clinical scanners in commercial production today produce magnetic fields at strengths of 1.5 or 3.0 tesla (T).
When the patient is placed in the MRI scanner, the magnetic dipoles in the tissues line up relative to the external magnetic field. Some dipoles will point in the direction of the external field (“north”), some will point in the opposite direction (“south”), but the net magnetization vector of the dipoles (the sum of individual spins) will point in the direction of the external field (“north”), and this will be the tissue’s acquired net magnetization. At this point, a small proportion of the protons (and therefore the net magnetization vector of the tissue) is aligned along the external field (longitudinal magnetization), and the protons precess with a certain frequency. The term precession describes a proton spinning about its own axis and its simultaneous wobbling about the axis of the external field (see Fig. 33A.3, B). The frequency of precession is directly proportional to the strength of the applied external magnetic field.
As a next step in obtaining an image, a radiofrequency pulse is applied to the part of the body being imaged. This is an electromagnetic wave, and if its frequency matches the precession frequency of the protons, resonance occurs. Resonance is a very efficient way to give or receive energy. In this process, the protons receive the energy of the applied radiofrequency pulse. As a result, the protons flip, and the net magnetization vector of the tissue ceases transiently to be aligned with that of the external field but flips into another plane, thereby transverse magnetization is produced. One example of this is the 90-degree radiofrequency pulse that flips the entire net magnetization vector by 90 degrees to the transverse (horizontal) plane (Fig. 33A.4). What we detect in MRI is this transverse magnetization, and its degree will determine the signal intensity. Through the process of electromagnetic induction, rotating transverse magnetization in the tissue induces electrical currents in receiver coils, thus accomplishing signal detection. Several cycles of excitation pulses by the scanner with detection of the resulting electromagnetic signal from the imaged subject are repeated per imaged slice. This occurs while varying two additional magnetic field gradients along the x and y axes for each cycle. Varying the magnetic field gradient along these two additional axes, known as phase and frequency encoding, is necessary to obtain sufficient information to decode the spatial coordinates of the signal emitted by each tissue voxel. This is accomplished using a mathematical algorithm known as a Fourier transform. The final image is produced by applying a gray scale to the intensity values calculated by the Fourier transform for each voxel within the imaging plane, corresponding to the signal intensity of individual tissue elements.
T1 and T2 Relaxation Times
During the process of resonance, the applied 90-degree radiofrequency pulse flips the net magnetization vectors of the imaged tissues to the transverse (horizontal) plane by transmitting electromagnetic energy to the protons. The radiofrequency pulse is brief, and after it is turned off the magnitude of the net magnetization vector starts to decrease along the transverse or horizontal plane and return (“recover or relax”) toward its original position, in which it is aligned parallel to the external magnetic field. The relaxation process, therefore, changes the magnitude and orientation of the tissue’s net magnetization vector. There is a decrease along the horizontal or transverse plane and an increase (recovery) along the longitudinal or vertical plane (Fig. 33A.5).
To understand the meaning of T1 and T2 relaxation times, the decrease in the magnitude of the horizontal component of the net magnetization vector and its simultaneous increase in magnitude along the vertical plane should be analyzed independently. These processes are in fact independent and occur at two different rates, T2 relaxation always occurring more rapidly than T1 relaxation (Fig. 33A.6). The T1 relaxation time refers to the time required by protons within a given tissue to recover 63% of their original net magnetization vector along the vertical or longitudinal plane immediately after completion of the 90-degree radiofrequency pulse. As an example, a T1 time of 2 seconds means that 2 seconds after the 90-degree pulse is turned off, the given tissue’s net magnetization vector has recovered 63% of its original magnitude along the vertical (longitudinal) plane. Different tissues may have quite different T1 time values (T1 recovery or relaxation times). T1 relaxation is also known as spin-lattice relaxation.
Repetition Time and Time to Echo
As mentioned before, the amount of the signal detected by the receiver coils depends on the magnitude of the net magnetization vector along the transverse or horizontal plane. Using certain operator-dependent parameters, it is possible to influence how much net magnetization strength (in other words, vector length) will be present in the transverse plane for the imaged tissues at the time of signal acquisition. During the imaging process, the initial 90-degree pulse flips the entire vertical or longitudinal magnetization vector into the horizontal plane. When this initial pulse is turned off, recovery along the longitudinal plane begins (T1 relaxation). Subsequent application of a second radiofrequency pulse at a given time after the first pulse will flip the net magnetization vector that recovered so far along the longitudinal plane back to the transverse plane. As a result, we can measure the magnitude of the net longitudinal magnetization that had recovered within each voxel at the time of application of the second pulse, provided that signal acquisition is begun immediately afterwards. The time between these radiofrequency pulses is referred to as repetition time, or TR (Fig. 33A.7). It is important to realize that contrary to the T1 and T2 times, which are properties of the given tissue, the repetition time is a controllable parameter. By selecting a longer TR, for instance, we allow more time for the net magnetization vector to recover before we flip it back to the transverse plane for measurement. A longer TR, because it increases the amount of signal that can potentially be detected, will also result in a higher signal-to-noise ratio, with higher image quality.
Tissue Contrast (T1, T2, and Proton Density Weighting)
By using various TR and TE values, it is possible to increase (or decrease) the contrast between different tissues in an MR image. Achieving this contrast may be based on either the T1 or the T2 properties of the tissues in conjunction with their proton density. Selecting a long TR value reduces the T1 contrast between tissues (Fig. 33A.8). Thus, if we wait long enough before applying the second 90-degree pulse, we allow enough time for all tissues to recover most of their longitudinal or vertical magnetization. Because T1 is relatively short, even for tissues with the longest T1, this is possible without resulting in excessively long scan times. Since after a long TR, the longitudinally oriented net magnetization vectors of separate tissue types are all of similar magnitudes prior to being flipped into the transverse plane by the second pulse, a long TR will result in little T1 tissue contrast. Conversely, by selecting a short TR value, there will be significant variation in the extent to which tissues with different T1 relaxation times will have recovered their longitudinal magnetization prior to being flipped by the second 90-degree pulse (see Fig. 33A.8). Therefore, with a short TR, the second pulse will flip magnetization vectors of different magnitudes into the transverse plane for measurement, resulting in more T1 contrast between the tissues.
During T2 relaxation in the transverse plane, selecting a short TE will give higher measured signal intensities (as a short TE will not allow enough time for significant dephasing, i.e., transverse magnetization loss), but tissues with different T2 relaxation times will not show much contrast (Fig. 33A.9). This is because by selecting a short time until measurement (short TE) we do not allow significant T2-related magnitude differences to develop. If we select longer TE values, tissues with different T2 relaxation times will have time to lose different amounts of transverse magnetization, and therefore by the time of signal measurement, different signal intensities will be measured from these different tissues (see Fig. 33A.9). This is referred to as T2 contrast.
On T1-weighted images, substances with a longer T1 relaxation time (such as water) will be darker. This is because the short TR does not allow as much longitudinal magnetization to recover, so the vector flipped to the transverse plane by the second 90-degree pulse will be smaller with a lower resulting signal strength. Conversely, tissues with shorter T1 relaxation times (such as fat or some mucinous materials) will be brighter on T1-weighted images, as they recover more longitudinal magnetization prior to their proton spins being flipped into the transverse plane by the second 90-degree pulse (Fig. 33A.10). Among many other applications of T1-weighted images, they allow for evaluation of BBB breakdown: areas with abnormally permeable BBB show increased signal after the intravenous administration of gadolinium. Gadolinium administration is contraindicated in pregnancy. Breast-feeding immediately after receiving gadolinium is generally regarded to be safe (Chen et al., 2008). Renally impaired patients are susceptible to an uncommon but serious adverse reaction to gadolinium, nephrogenic systemic fibrosis (Marckmann et al., 2006).
On T2-weighted images, substances with longer T2 relaxation times (e.g., water) will be brighter because they will not have lost as much transverse magnetization magnitude by the time the signal is measured (Fig. 33A.11). The T1 and T2 signal characteristics of various tissues or substances found in neuroimaging are listed in Table 33A.1.
Table 33A.1 MRI Signal Intensity of Some Substances Found in Neuroimaging
T1-Weighted Image | T2-Weighted Image | |
---|---|---|
Air | ↓ ↓ ↓ ↓ | ↓ ↓ ↓ ↓ |
Free water/CSF | ↓ ↓ ↓ | ↑ ↑ ↑ |
Fat | ↑ ↑ ↑ | ↑ |
Cortical bone | ↓ ↓ ↓ | ↓ ↓ ↓ |
Bone marrow (fat) | ↑ ↑ | ↑ |
Edema | ↓ | ↑ ↑ |
Calcification | ↓ (Heavy amounts of Ca++) ↑ (Little Ca++, some Fe+++) | ↓ |
Mucinous material | ↑ | ↓ |
Gray matter | Lower than in T2-WI | |
White matter | Higher than in T2-WI | |
Muscle | Similar to gray matter | Similar to gray matter |
Blood products: | ||
• Oxyhemoglobin | Similar to background | ↑ |
• Deoxyhemoglobin | ↓ | ↓ |
• Intracellular methemoglobin | ↑ ↑ | ↓ |
• Extracellular methemoglobin | ↑ ↑ | ↑ ↑ |
• Hemosiderin | ↓ | ↓ ↓ ↓ |
CSF, Cerebrospinal fluid; MRI, magnetic resonance imaging; T2-WI, T2-weighted image.
Magnetic Resonance Image Reconstruction
To construct an MR image, a slice of the imaged body part is selected, then the signal coming from each of the voxels making up the given slice is measured. Slice selection is achieved by setting the external magnetic field to vary linearly along one of the three principal axes perpendicular to the axial, sagittal, and coronal planes of the subject being imaged. As a result, protons within the slice to be imaged will precess at a Larmor frequency different from the Larmor frequency within all other imaging planes perpendicular to the axis along which the magnetic field gradient is applied. The Larmor frequency is the natural precession frequency of protons within a magnetic field of a given strength and is calculated simply as the product of the magnetic field, B0, and the gyromagnetic ratio, gamma. The precession frequency of a hydrogen proton is therefore directly proportional to the strength of the applied magnetic field. The gyromagnetic ratio for any given nucleus is a constant, with a value for hydrogen protons of 42.58 MHz/T. In slices at lower magnetic strengths of the gradient, the protons precess more slowly, whereas in slices at higher magnetic field strengths, the protons precess more quickly. Based on the property of nuclear magnetic resonance, the applied radiofrequency pulse (which flips the magnetization vector to the transverse plane) will stimulate only those protons with a precession frequency that matches the frequency of the applied radiofrequency pulse. By selecting the frequency of the stimulating radiofrequency pulse during the application of the slice selection gradient, we can choose which protons (those with a specific Larmor frequency) to stimulate (“make resonate”), and thereby we can select which slice of the body to image (Fig. 33A.12).
In the online version of this chapter (available at www.expertconsult.com), there is a discussion of the nature and application of the following MRI sequences or techniques: spin echo and fast (turbo) spin echo; gradient-recalled echo (GRE) sequences, partial flip angle; inversion recovery sequences (FLAIR, STIR); fat saturation; echoplanar imaging; diffusion-weighted magnetic resonance imaging (DWI); perfusion-weighted magnetic resonance imaging (PWI); susceptibility-weighted imaging (SWI); diffusion tensor imaging (DTI); and magnetization transfer contrast imaging.
Magnetic Resonance Imaging
Inversion Recovery Sequences (FLAIR, STIR)
Inversion recovery techniques use a unique pulse sequence to avoid signal detection from the selected tissues (fat or CSF). Initially, a 180-degree radiofrequency pulse is applied. This will flip the longitudinal magnetization vectors of all tissues by 180-degrees, so that the vectors will point downward (south). Next, the flipped vectors are allowed to start recovering according to their respective T1 times. As the downward-pointing vectors recover, they become progressively smaller, eventually reaching zero magnitude, and from that point they start growing and pointing upward (north). Without interference, they recover the original longitudinal magnetization. However, during the process of recovery, after a time period referred to as inversion time (TI), a 90-degree pulse is applied. This will flip the longitudinal vectors to the transverse plane, where signal detection occurs. The amount of magnetization flipped by this pulse depends on how far the longitudinal recovery has been allowed to proceed. If the 90-degree pulse is applied when a given tissue’s vector happens to be zero (this is the so-called null point), no magnetization will be flipped from that tissue to the transverse plane, and therefore no signal will be detected from that tissue. Different tissues recover their longitudinal magnetization at different rates according to their specific T1 times. Knowing a given tissue’s T1 time, we can calculate when it will reach the null point (when its longitudinal magnetization is zero), and if we apply the 90-degree pulse at that point, we will not detect any signal from that particular tissue. The inversion time is linearly dependent upon a given tissue’s T1 value, being calculated as 0.69 multiplied by the T1 value. In the FLAIR (fluid-attenuated inversion recovery) sequence, the inversion time (when the 90-degree pulse is applied) occurs when the magnetization vector for the CSF is at the null point, so no signal will be detected from the CSF (Fig. 33A.13). In FLAIR images, the dark CSF is in sharp contrast with the hyperintensity of PV lesions, allowing their better identification. In STIR (short TI, or tau inversion, recovery) imaging, which is a fat-suppression technique, the methodology is essentially the same as for FLAIR. However, instead of CSF, the signal from fat is nulled. The TI for the STIR technique is set to 0.69 times the T1 of fat, which results in application of the final 90-degree pulse when the fat tissue’s magnetization is at the null point, so no signal from fat will be detected.
Fat Saturation
Fat saturation is a pulse sequence used to suppress the bright signal of adipose tissue and thereby allow better visualization of hyperintense abnormalities or, upon gadolinium administration, abnormal enhancement that otherwise may be obscured by fatty tissue in areas such as the orbits or spinal epidural space. In the same external magnetic field, the protons in fat versus water experience slightly different local magnetic fields because of differences in molecular structure. As a consequence, the protons in the fat will have a slightly different precession frequency from that of the water protons and will therefore resonate with a slightly different externally applied pulse frequency. Thus, it is possible to apply a radiofrequency pulse (presaturation pulse) that will resonate selectively with the fat-based protons only. This pulse will flip the magnetization vector of fat to the transverse plane, where it will be destroyed or “spoiled” by a gradient pulse. Next, the planned pulse sequence is applied, and at that point the obtained transverse magnetization will not have the component from fat, as it was destroyed (Fig. 33A.14). Therefore, by the time of TE, no signal will be detected from the fat tissue, and areas of fat will be dark in the image, allowing hyperintense enhancement to stand out.
Susceptibility-Weighted Imaging
SWI (Haacke et al., 2009; Mittal et al., 2009) uses a high spatial resolution 3D gradient echo imaging sequence. The contrast achieved by this sequence distinguishes the magnetic susceptibility difference between oxygenated and deoxygenated hemoglobin. Since the applied phase postprocessing sequence accentuates the paramagnetic properties of deoxyhemoglobin and blood degradation products such as intracellular methemoglobin and hemosiderin, this technique is very sensitive for intravascular venous deoxygenated blood as well as extravascular blood products. It has been used for evaluation of venous structures, hence the earlier name high-resolution blood oxygen level–dependent venography, but the clinical application is now much broader. Its exquisite sensitivity for blood degradation products makes this technique very useful when evaluating any lesion (e.g., stroke, AVM, cavernoma or neoplasm) for associated hemorrhage (Fig. 33A.15). It is also used for imaging microbleeds associated with traumatic brain injury, diffuse axonal injury, or cerebral amyloid angiopathy.
Diffusion Tensor Imaging
Diffusion tensor imaging is a more advanced type of diffusion imaging capable of quantifying anisotropy of diffusion in white matter. Diffusion is isotropic when it occurs with the same intensity in all directions. It is anisotropic when it occurs preferentially in one direction, as along the longitudinal axis of axons. For this reason, DTI finds its greatest current application in MRI examinations of the white matter. As opposed to characterizing diffusion within each voxel with just a single apparent diffusion coefficient, as in DWI, in DTI intravoxel diffusion is measured along three, six, or more gradient directions. The measured values and their directions are called eigenvectors. The vector that corresponds to the principal direction of diffusion (the direction in which diffusion is greatest in magnitude) is called the principal eigenvector. In normal white matter, diffusion anisotropy is high because diffusion is greatest parallel to the course of the nerve fiber tracts. Therefore, the principal eigenvector delineates the course of a given nerve fiber pathway. Diffusion tensor images can be displayed as maps of the principal eigenvectors which will show the direction/course of the given white matter tract (tractography). These images can also be color coded, allowing for more spectacular visualization of nerve fiber tracts (Fig. 33A.16). Any disruption of a given nerve fiber tract (e.g., MS, trauma, gliosis) will reduce anisotropy, and the disruption of the white matter tract can be visualized. Tensor imaging/tractography is useful in imaging of degenerating white matter tracts and also in surgical resection planning, when the anatomical relationship of the resectable lesion and the adjacent fiber tracts has to be evaluated to avoid or reduce surgical injury to critical pathways.
Magnetization Transfer Contrast Imaging
As the name indicates, magnetization transfer contrast imaging is a technique that produces increased contrast within an MR image, specifically on T1-weighted gadolinium-enhanced images and in magnetic resonance angiography (Henkelman et al., 2001). In water, hydrogen atoms are relatively loosely bound to oxygen atoms, and they move frequently between them, binding to one oxygen atom then switching to another. In other tissues (e.g., lipids, proteins), the hydrogen atoms are more tightly bound and tend to stay in one place for longer periods of time. Nevertheless, it does happen that a “bound” hydrogen in lipid or protein is exchanged with a “more free” hydrogen from water. In magnetization transfer imaging, at the beginning of the sequence a radiofrequency pulse is applied that saturates the bound protons in lipids and proteins but does not affect the free protons in water. In regions where magnetization transfer (i.e., exchange of saturated protons with free protons) occurs, the saturated protons will decrease the signal obtained from the imaged free protons. The more frequently this magnetization transfer occurs, the less signal is obtained from the region and the darker the region will be in the image. Magnetization transfer happens more frequently in the white matter, resulting in signal loss, and therefore on magnetization transfer images, the white matter appears darker. The CSF on the other hand, where magnetization transfer does not occur, does not lose signal. Magnetization transfer is minimal in blood because of the high amount of free water protons.
Structural Neuroimaging in the Clinical Practice of Neurology
Brain Diseases*
Brain Tumors
Epidemiology, pathology, etiology, and management of cancer in the nervous system are discussed in Chapter 52A, Chapter 52B, Chapter 52C, Chapter 52D, Chapter 52E, Chapter 52F, Chapter 52G . From the standpoint of structural neuroimaging, a useful anatomical classification distinguishes two main groups: intraaxial and extraaxial tumors. Intraaxial tumors are within the brain parenchyma, extraaxial tumors are outside the brain parenchyma (involving the meninges or, less commonly, the ventricular system). Intraaxial tumors are usually infiltrative with poorly defined margins. Conversely, extraaxial tumors, even though they often compress or displace the adjacent brain, are usually demarcated by a cerebrospinal (CSF) cleft or another tissue interface between tumor and brain parenchyma. For differential diagnostic purposes, intraaxial primary brain neoplasms can be further divided into the anatomical subgroups of supratentorial and infratentorial tumors (Table 33A.2).
Intraaxial Primary Brain Tumors
Ganglioglioma and Gangliocytoma
On MRI (Provenzale et al., 2000) the solid component is usually isointense on T1 and hypo- to hyperintense on T2-weighted images. The cystic component, if present, exhibits CSF signal characteristics. The associated mass effect is variable. With contrast, various enhancement patterns are seen—homogenous or rim pattern—but no enhancement is also possible.
Pilocytic Astrocytomas
Pilocytic astrocytomas have two major groups: juvenile and adult. These tumors are classified as WHO grade I. Juvenile pilocytic astrocytomas are the most common posterior fossa tumors in children. The most common locations are the cerebellum, at the fourth ventricle, third ventricle, temporal lobe, optic chiasm, and hypothalamus (Koeller and Rushing, 2004). The appearance is often lobulated, and the lesion appears well demarcated on MRI. Hemorrhage and necrosis are uncommon. Areas of calcification may be present. The tumor usually exhibits solid as well as cystic components, with or without a mural nodule. The adult form is usually well circumscribed, often calcified, and typically exhibits a large cyst with a mural nodule. On MRI, the solid portions of the tumor are iso- to hypointense on T1 and iso- to hyperintense on T2-weighted images (Arai et al., 2006). The cystic component usually exhibits CSF signal characteristics. The associated edema and mass effect is usually mild, sometimes moderate. With gadolinium, the solid components (including the mural nodule) enhance intensely, but not the cyst, which rarely may show rim enhancement.
Pleomorphic Xanthoastrocytoma
Pleomorphic xanthoastrocytoma is a rare variant of astrocytic tumors. It is thought to arise from the subpial astrocytes and typically affects the cerebral cortex and adjacent meninges and may cause erosion of the skull. The most common location is the temporal lobe. It is classified as WHO grade II. It usually occurs in the second and third decades of life, and patients often present with seizures. On MRI (Tien et al., 1992) usually a well-circumscribed cystic mass appears in a superficial cortical location. A solid portion or mural nodule is often seen, and the differential diagnosis includes pilocytic astrocytoma and ganglioglioma. The signal characteristics are hypointense or mixed on T1, and hyperintense or mixed on T2-weighted images. With contrast, the solid portions and sometimes the adjacent meninges enhance. Calcification may be present. There is mild or no mass effect associated with this tumor.
Low-Grade Astrocytomas
Fibrillary astrocytomas, also termed diffuse astrocytomas, represent approximately 10% of all gliomas. Low-grade (WHO grade I and II) astrocytomas belong to this group (Figs. 33A.17 and 33A.18). These are well-differentiated tumors, usually arising from the fibrillary astrocytes of the white matter. Even though imaging may show a fairly well-defined boundary, these tumors are infiltrative and usually spread beyond their macroscopic border. Two-thirds of cases are supratentorial. A subgroup of these astrocytomas involves specific regions such as the optic nerves/tracts or the brainstem.
Oligodendroglioma
Oligodendroglioma accounts for 5% to 10% of all gliomas. It arises from the oligodendroglia that form the myelin sheath of the central nervous system (CNS) pathways. Oligodendroglioma occurs most commonly in young and middle-aged adults, with a median age of onset within the fourth to fifth decades and a male predominance of up to 2 : 1. Seizure is often the presenting symptom. The most common location is the supratentorial hemispheric white matter, and it also involves the cortical mantle. The tumor often has cystic components and at least microscopically, in 90% of cases also shows calcification. Hemorrhage and necrosis are rare, and the mass effect is not impressive. On MRI (Koeller and Rushing, 2005) the appearance is heterogenous, and the tumor is hypo- and isointense on T1 and hyperintense on T2. With gadolinium, the enhancement is variable, usually patchy, and the periphery of the lesion tends to enhance more intensely. Oligodendrogliomas are hypercellular and have been noted to appear hyperintense on diffusion-weighted images (Fig. 33A.19).
< div class='tao-gold-member'>
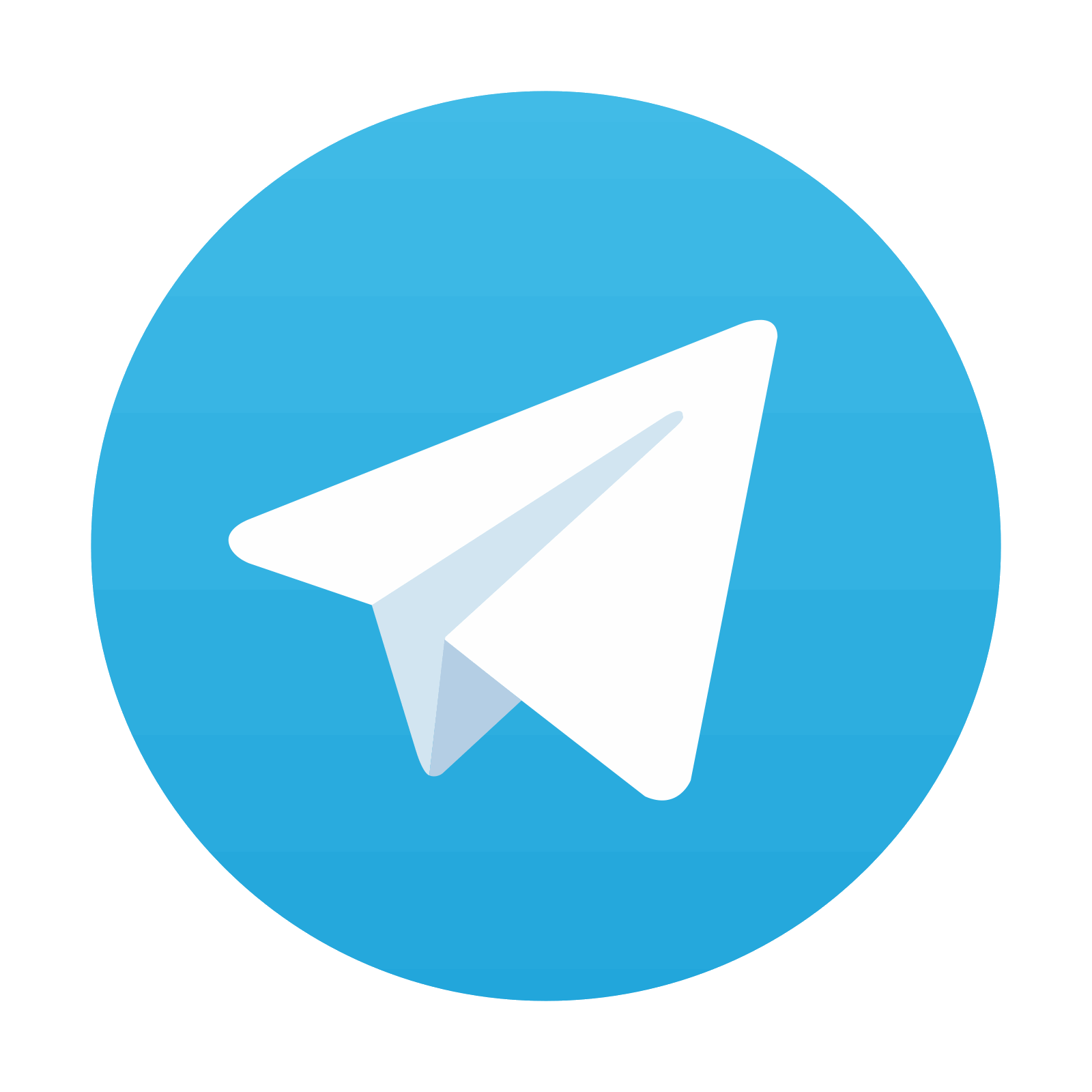
Stay updated, free articles. Join our Telegram channel
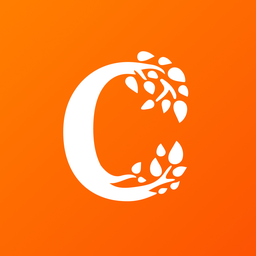
Full access? Get Clinical Tree
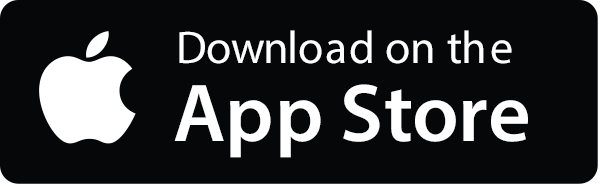
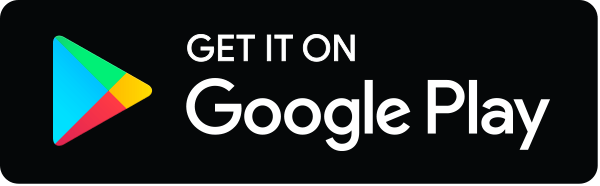