Abstract
Mammalian spinal networks have sophisticated neural networks that intrinsically contain the necessary information to generate locomotion, and these central pattern-generating networks are viable targets for recovery after spinal cord injury. Patterned locomotor activity was shown to be driven by constant epidural stimulation in humans when in lying positions, indicating the intrinsic capacity of the human spinal cord interneuronal systems to generate oscillatory and extensor patterns. Epidural stimulation of the lumbosacral spinal cord uses the capacity of the intact spinal networks below the level of injury to drive plasticity and enhance the excitatory drive of the spinal cord, and has promise as a new clinical tool.
Keywords
Neural networks, Programming, Spinal cord epidural stimulation, Spinal cord injury, Standing, Stepping, Voluntary movement
Outline
Historical Perspective 1183
Spinal Cord Epidural Stimulation Facilitates Stepping and Standing in Mammals 1184
Evidence of Sophisticated Neural Networks in the Human Spinal Cord Circuitry 1184
Spinal Cord Epidural Stimulation Facilitates Standing and Stepping Patterns in Humans 1185
Spinal Cord Epidural Stimulation Evoked Unforeseen Voluntary Movement Responses and Independent Standing in Humans With Motor-Complete Spinal Cord Injury 1185
Rationale for Neuromodulation Target Selection and Approach 1186
Programming and Other Points of Consideration 1186
Voluntary Movement 1187
Selection of Epidural Stimulation Parameters for Promoting Voluntary Leg and Trunk Movement 1187
Role of Activity-Based Training With Spinal Cord Epidural Stimulation for Recovery of Motor Function for Voluntary Leg and Trunk Movements 1188
Standing 1188
Selection of Epidural Stimulation Parameters for Promoting Standing 1188
Role of Activity-Based Training With Spinal Cord Epidural Stimulation for the Recovery of Motor Function for Standing 1191
Stepping 1191
Selection of Epidural Stimulation Parameters for Promoting Standing 1192
Role of Activity-Based Training With Spinal Cord Epidural Stimulation for Recovery of Motor Function for Step Training 1192
Summary and Future Directions 1193
References 1193
Historical Perspective
Over a century of elegant experiments have culminated in demonstrating that mammalian spinal networks have sophisticated neural networks which intrinsically contain the necessary information to generate locomotion, and these central pattern-generating networks are viable targets for recovery after spinal cord injury ( ). Coordinated locomotor activity in mammals launched six decades of detailing how these interneuronal systems function, and their interactions with sensory information and descending systems to generate effective motor output for postural and locomotor behavior ( ). Postural reflexes in spinal rabbits and a myriad of balance experiments in cats also identified spinal mechanisms for postural control in the mammalian spinal cord ( ). Skepticism continued as to whether these mechanisms were conserved in the human spinal cord, and their utility for recovery in paralysis is still challenged today. Yet these historical experiments led to the exploration of the response of the human spinal circuitry to neuromodulation using epidural stimulation in the lumbosacral region of the spinal cord for recovery of paralysis.
Spinal Cord Epidural Stimulation Facilitates Stepping and Standing in Mammals
Epidural stimulation in mammalian complete transection models showed the transformation of nonfunctional spinal circuits into functional locomotor states and also enabled processing of sensory information related to loading and position during stepping without descending influence ( ). Spinal epidural stimulation in decerebrate cats generated locomotor patterns modulation by peripheral feedback ( ). A series of studies on animal models with complete spinal cord injury suggested that the spinal cord contains the neural networks that control balance, and that these networks are normally activated by a tonic supraspinal drive ( ). Spinal cord epidural stimulation (scES) facilitated postural responses in these animal models. Furthermore, the fact that postural control was recovered to some extent after the suppression of vestibular, visual, and head–neck–trunk sensory input implies that somatosensory information from the lower limbs plays a crucial role in generating appropriate postural responses ( ). Interestingly, postural training and pharmacological interventions enhanced the positive effects of scES on postural control; however, even the combination of these three interventions was not sufficient to regain normal postural functions ( ).
Evidence of Sophisticated Neural Networks in the Human Spinal Cord Circuitry
Patterned locomotor activity was shown to be driven by constant epidural stimulation in humans when in lying positions, indicating the intrinsic capacity of the human spinal cord interneuronal systems to generate oscillatory and extensor patterns in the absence of observable descending drive and those sensory cues associate with weight-bearing loading ( ). Numerous human experiments also demonstrated that individuals who by clinical and functional assessments could not move voluntarily below their injury did show complex motor patterns when carefully assessed by clinical ( ) and neurophysiological measures ( ), and in some cases these were modulated with sensory cues ( ) and intentional efforts ( ). These indirect human experiments were the closest results to those reflecting the most definitive experiments in mammals of fictive locomotion, detailing the capacity of these circuits to control locomotion by integration of complex sensory signals ( ).
The human spinal cord was also shown to generate locomotor patterns and weight-bearing steps successfully in the absence of input from the brain when provided with specific and appropriate sensory information related to locomotion ( ), as shown in other mammals. This experimental approach was translated into an activity-based therapy, locomotor training, with significant success for postural and walking recovery in those with incomplete spinal cord injury ( ). Individuals with very low levels of neuromuscular activity, including those with limited or no detectable voluntary activity below the injury, recovered significant postural stability and nonweight-bearing mobility, but were not able to generate independent steps over ground. These experiments indicated sensory information was modulated during stepping even in individuals with motor-complete spinal cord injury, and raised the realistic possibility that epidural stimulation would enable the circuitry to generate locomotor patterns.
Spinal Cord Epidural Stimulation Facilitates Standing and Stepping Patterns in Humans
A series of studies performed on individuals with motor-complete spinal cord injury who were implanted with an epidural stimulation unit with the intent to reduce spasticity showed that lower-limb extensor motor patterns could be elicited in the supine position by scES ( ). In particular, two active electrodes of a quadripolar array, implanted at a vertebral level ranging from T12 to L1, were used to stimulate the spinal cord by applying stimulation amplitudes well above motor threshold at frequencies ranging from 5 to 15 Hz. These stimulation parameters initiated and retained lower-limb extension that resulted from the greater activation of hamstring and triceps surae muscles compared to the quadriceps and tibialis anterior, respectively. The same authors reported that the motor pattern became rhythmic when the stimulation frequency was increased to 21–31 Hz, without any change in stimulation site and amplitude. These interesting results were interpreted to mean that different stimulation frequencies at the same site of stimulation would access different inhibitory and/or excitatory pathways within spinal networks to elicit different motor patterns (i.e., tonic or rhythmic). Thus at frequencies between 5 and 15 Hz the spinal network was conceivably configured via presynaptic and synaptic mechanisms to favor the electromyographic (EMG) “extensor pattern,” and at higher frequencies to favor a rhythmic locomotor-like pattern. However, no recovery of stepping or standing ability was reported using these relatively higher- and lower-frequency stimulation paradigms.
The concept of implanting specifically for standing and stepping and integrating the ability of the spinal cord circuitry to interpret sensory cues for standing and stepping was tested in a motor-complete but sensory-incomplete individual with chronic spinal cord injury by implanting an epidural stimulator over the L1–S1 spinal cord. This individual achieved full weight-bearing standing with only assistance for balance when using scES parameters specific for standing (Stand-scES) and bilateral load-bearing proprioceptive input. Locomotor patterns that were not observed in 170 sessions of locomotor training emerged only with scES using parameters specific for stepping (Step-scES) and proprioceptive input related to alternating bilateral loading and kinematic extension and flexion. Tonic epidural stimulation modulated the human spinal circuitry into a physiological state that enabled sensory input, derived from standing and stepping movements, to serve as a source of neural control to perform these critical motor tasks lost after severe spinal cord injury.
Spinal Cord Epidural Stimulation Evoked Unforeseen Voluntary Movement Responses and Independent Standing in Humans With Motor-Complete Spinal Cord Injury
Voluntary initiated movement generated by this individual with a motor-complete spinal cord injury observed after 4 months of continuous scES revealed unexpected potential for even more recovery for motor control than thought possible by the original theory of the mammalian spinal cord containing intrinsic circuits only for repetitive locomotor-like patterns ( ). There may be the ability to target specific movements more precisely, generate multiple repetitions of movement, increase force, and sustain longer contractions evolved over time with task-specific training ( ). An initial interpretation of these unexpected observations was that the presence of ascending sensory fibers, albeit impaired, and significant neural plasticity driven by intense stimulation over several months had reconfigured sensory pathways into novel functional motor pathways allowing voluntary movement. In combination with scES configurations specific for voluntary movements (Vol-scES) and task-specific training, these pathways strengthened with further supraspinal and spinal plasticity. This hypothesis was addressed by testing an individual with a motor- and sensory-complete spinal cord injury. Most surprisingly, during his first attempts with Vol-scES (and with less than a week of any scES and no training) he was able to generate voluntary movements. Voluntary movements were also successful in the next two individuals, one with a motor-complete and sensory-incomplete spinal cord injury and the other with a motor- and sensory-complete spinal cord injury. The possibility of subliminal pathways still available for neural activation even in the most severe injuries now needs to be considered. Also, the theory of human control of voluntary movements attributed mostly or entirely to corticospinal mechanisms ( ), with the spinal cord as simply a conduit of these commands, may not be plausible in the context of these most recent findings in these four individuals with the most severe motor spinal cord injury.
Standing with full weight bearing and without physical assistance also improved with specific configurations for Stand-scES and repetitive daily training ( ). The human spinal circuitry generated motor patterns effective for standing without clinical or neurophysiological evidence of functional supraspinal connections. Sensory- and motor-complete spinal cord injury individuals achieved full weight-bearing standing without any external assistance and with minimal self-balance assistance only when the lumbosacral spinal cord was electrically stimulated. Stand-scES enabled independent standing and modulated lumbosacral spinal networks to a functional state that optimized the integration of task-specific afferent input to generate effective motor patterns with overall continuous EMG patterns in the lower-limb muscles and a constant level of ground reaction forces.
Rationale for Neuromodulation Target Selection and Approach
Neuromodulation for using epidural stimulation of the lumbosacral spinal cord following spinal cord injury remains an experimental strategy. The focus of most studies has been on individuals who have suffered a traumatic (nonprogressive) injury. Subjects have been at least 1 year after injury and have no significant comorbidities unassociated with the spinal cord injury. Antispasticity medications have been avoided for epidural stimulation, as they are presumed to inhibit modulation of the same synapses that are being targeted in the spinal networks ( ). Individuals with lower motor neuron injuries were not selected in these early experimental studies for epidural stimulation, as this injury is likely more complex and directly involves central pattern-generating interneurons, loss of supraspinal influence, and damage to the peripheral neurons. Thus early assessment of the potential for scES is for now being addressed in individuals with upper-thoracic and cervical injuries. Any stabilizing instrumentation below T8 might interfere with the ability to perform a laminectomy and accurately place the stimulating electrode array. However, future studies in those with lower-thoracic injuries will be important, especially given the realization that previously undetected spared supraspinal and spinal connections remain even after severe injury. Regardless of level of injury, the electrode is implanted at T11–T12 vertebral level, to maximize the circuitry potential of the lumbosacral spinal cord (L1–S1). The electrode lead placement is guided using motor-evoked potentials to minimize left/right physiological difference and fine-tune the position with respect to each individual’s physiology.
Programming and Other Points of Consideration
The epidural stimulation array implanted specifically for motor behavior was placed at spinal level T11–L1, and included 16 electrodes that can be set as cathodes, anodes, or inactive ( ). This capability leads to over 40 million different electrode configurations that can potentially be tested. The guidelines used to narrow down the number of potentially effective electrode configurations were derived from both previous findings reported in the literature and extensive neurophysiological mapping in the supine position ( ). Similarly, the effects of unbalanced anodes and cathodes between the lateral columns of the electrode array were tested, with the intent to alter the side-specific limb muscle recruitment if activation differences between the left and right lower limbs were present. In addition, mapping studies were performed with the individuals in supine position to identify the electrodes for specific motor pools. These guidelines were followed to adjust stimulation parameters during periodic assessments of EMG and behavioral patterns to optimize the desired motor behavior.
Our approach to scES for the recovery of function is to provide tonic subthreshold stimulation to specific motor pools to access the capacity of these networks. Then sensory cues and descending intentional commands are optimized to result in the desired motor behavior. Stimulation configurations (electrode anode and cathode selection, amplitude, frequency, and pulse width) are activity dependent and individualized to the neurophysiological profile of each participant. The first step in understanding individual responses is to perform detailed mapping of the motor responses relative to spatial characteristics of the stimulation profile lying supine at rest. The “mapping” of motor-evoked responses is achieved by stimulating at 2 Hz using adjacent bipolar configurations (local configurations) along the different columns of the electrode array. Although 2 Hz likely results in a slightly more complex response than a simple motor-evoked potential, we use this frequency rather than a lower one (e.g., 0.3 Hz would be physiologically less likely to result in inhibition) because the number of configurations that are needed to provide sufficient information would take an unreasonable number of hours to complete. Stimulation is ramped up at 0.1 V until activation threshold for all muscles is achieved. Once all muscles of the lower extremity are active, stimulation amplitude is increased by 0.5 V until a maximum of 10 V or the maximum tolerated by the individual, whichever is lowest. Activation thresholds for each muscle of interest and recruitment curves from peak-to-peak values of motor-evoked responses relative to stimulation amplitude are analyzed relative to spatial orientation in the electrode array. In addition to voltage response mapping, frequency response analysis is performed using selected electrode configurations and stimulation amplitudes. Then using six electrodes, three rostral and three caudal (global configuration), voltage and frequency responses are conducted. The information gained for the frequency response profile is used to determine the frequency–voltage combinations in which bursting versus tonic patterns can be driven by stimulation alone. This mapping offers the initial point for the selection of stimulation parameters for standing, voluntary activity, and stepping. Further mapping is done during the desired motor behavior to optimize for voluntary movement, standing and/or stepping, and individual differences.
There are currently significant limitations in available programming options, given these devices were designed primarily for the treatment of chronic pain for nonspinal cord injury populations. Limitations include the rate that different configurations can be delivered, the ability to change anode and cathode designations rapidly without a drop in voltage, a limit on varied frequencies and voltage delivery to electrodes, and software interface constraints. For long-term use the patient interface is difficult to manipulate, especially for those with hand function deficits. The requirement for the controller to be placed directly over the location on the body of the stimulator unit and held in place during changes limits the practical use of the voluntary and standing capabilities when in the home and the community. The lack of control algorithms also limits usability for daily activities of life. As we continue to understand the neurophysiological mechanisms and design better configurations and training paradigms, the technology will also need to be designed specifically for these applications and the spinal cord injury population with their physical limitations considered.
Voluntary Movement
The recovery of voluntary movement in individuals with motor-complete injury is dismal, with the only perceived potential treatment being neural repair or regeneration of corticospinal tracts ( ). The use of lumbosacral epidural stimulation for the execution of trunk and lower-extremity movements in motor-complete spinal cord injuries is a novel strategy that has been developing over the past few years. The concept of Vol-scES focuses on the capacity of the lumbosacral spinal cord to access intact networks below the injury and provide the appropriate selectivity of excitation to specific motor pools for the execution of the movement ( ). Vol-scES is designed to enable residual, otherwise undetectable, supraspinal pathways to relay intentional signals for specific movement.
Selection of Epidural Stimulation Parameters for Promoting Voluntary Leg and Trunk Movement
Vol-scES has to be individualized, specific, and targeted to a precise action. Selection of stimulation parameters (electrode anode and cathode selection, amplitude, frequency, and pulse width) to allow a voluntary movement of a single joint or multiple joints starts with mapping the motor responses to stimulation of various electrode combinations. Mapping is used to establish relationships between muscle thresholds and spatial orientation in the electrode array, as well as frequency–voltage relationships at various electrode combinations. This information is used to develop more complex electrode configurations that enable voluntary control. Configuration testing is performed repeatedly and synergistically modified until the optimal functional movement is achieved. Optimal Vol-scES configurations are set at subthreshold stimulation amplitudes. Stimulation frequencies have ranges from 25 to 60 Hz, dependent on the involuntary burst-generation frequency dependency for each individual. Individuals with higher levels of excitability are typically at the higher and lower ranges, making the interaction of stimulation frequency and amplitude a key component in the selection of the optimal Vol-scES configuration. Frequent reevaluation of Vol-scES configurations is critical to maintain an optimal interaction between scES and the state of the spinal cord networks. Continuous training promotes spinal cord plasticity, which then results in the need for parameter adjustments.
The first results of Vol-scES promoting the ability to execute voluntary movement of the lower extremities in individuals with motor-complete spinal cord injury have been published in recent years. Results from individuals implanted in the past 2 years show the ability to obtain Vol-scES parameters that promote voluntary movement of the lower extremities in individuals with injury levels ranging from C4 to T5. Evidence shows that return of voluntary control of single or multiple joint movements in the presence of Vol-scES did not require intensive training or practice. Following the optimization of Vol-scES parameters, individuals with a motor-complete spinal cord injury were able to move their lower extremities after years of complete paralysis. Fine motor control and motor pool selectivity were shown as important details in the execution of the movements.
Similar to lower-extremity movement, Vol-scES parameters are also optimized to target trunk musculature and promote voluntary engagement of key muscles during sitting activities. Cervical and high-thoracic spinal cord injuries result in paralysis of trunk muscles involved in the control of sitting posture ( ). A key component of noncompensatory sitting posture is the required tonic activation of trunk muscles, which are shown to be impaired after spinal cord injury. Currently the most popular optimized Vol-scES for trunk activation involves rostral configurations spanning one to two rows of the electrode array to promote the flow of current toward the thoracic levels of the spinal cord segments. Stimulation frequencies range from 25 to 30 Hz, while stimulation voltages are increased until anterior and posterior trunk muscles have low-level tonic activation to give the ability to stabilize and move the trunk. The use of Vol-scES during activities challenging posture and control while sitting promotes isolation and activation of stabilizing muscles while decreasing compensatory movements.
Role of Activity-Based Training With Spinal Cord Epidural Stimulation for Recovery of Motor Function for Voluntary Leg and Trunk Movements
Daily practice of voluntary movement promoted increases in fidelity of leg movement and a need for lower stimulation amplitude to generate the same or greater amount of force during the movement. Core activation following spinal cord injury is also translated to improved physical exercise capacity and endurance. Use of a Vol-scES trunk configuration by individuals with a high-thoracic motor-complete spinal cord injury during weight-lifting exercises has been reported to increase exercise efficacy (unpublished results). Similar to training and practice of lower-extremity movement, frequent use of Vol-scES for core exercises promotes spinal cord plasticity and has resulted in increased activation of rectus abdominus and erector spinae during some sitting tasks in the absence of Vol-scES.
Standing
The loss of standing ability is one of the many dramatic consequences of a severe spinal cord injury. When an individual with motor-complete spinal cord injury is assisted (by trainers) to perform a sitting-to-standing transition, lower-limb EMG activity is usually observed during the transition phase but little or no EMG activity is detected when the individual achieves the upright position, which can be maintained only with external assistance. Recently it has been demonstrated that the combination of scES and task-specific stand training can progressively promote the recovery of standing ability ( ). In fact, clinically complete spinal cord injury individuals were able to stand bearing their full bodyweight without any external assistance, and with minimal self-assistance for balance provided by their upper limbs.
Selection of Epidural Stimulation Parameters for Promoting Standing
This standing behavior was not achieved by eliciting a lower-limb extensor pattern by stimulation alone; rather, it was achieved by applying stimulation parameters that modulated the level of excitability of the lumbosacral spinal circuitry so the sensory information derived from the lower limbs (i.e., from muscle and cutaneous receptors) could be integrated by the spinal circuitry and used as a source of motor control to generate motor patterns effective for standing. This approach coincided with the selection of stimulation parameters that induced little or no lower-limb muscle activation while the individuals were relaxed in sitting position. In turn, EMG activity of lower-limb muscles was significantly modulated when the sitting-to-standing transition occurred and the loading of the legs was initiated. Importantly, the remarkable EMG pattern modulation occurred without any change in the stimulation parameters, as shown in Fig. 98.2 panels C and D. Motor patterns effective for stable standing involved the overall continuous (not rhythmic) activation of several lower-limb muscles that led to constant levels of ground reaction forces.



A series of experiments pointed out that the appropriate selection of stimulation amplitude, frequency, and electrode configuration is crucial to promote effective motor patterns for standing, and the appropriate stimulation parameters are specific to individuals ( ). Generally, cathodes located in the caudal region of the array, and more caudally than anodes, were more effective in facilitating a lower-limb extension pattern than stimulating the upper lumbar cord only or placing anodes more caudally than cathodes ( ). The effects of scES site on topographical motor pool recruitment were also taken into consideration. In particular, the electrode field was narrowed to the caudal portion of the electrode array or extended toward the rostral portion of the array to increase the excitability of distal or proximal muscles’ motor neuron pools, respectively. Generally, stimulation frequencies between 25 and 60 Hz were found effective to promote standing independent of external assistance. These higher frequencies conceivably promote the progressive contribution of somatosensory information from the lower limbs in shaping the evoked responses to spinal stimulation through the activation of interneurons. In fact, considering overall continuous EMG patterns, greater variability of the spinal-cord-evoked responses was observed at higher as compared to lower frequencies. Also, lower stimulation frequencies (i.e., <15 Hz) brought about pulsatile muscle contractions timed with the stimulation, which contributed to ineffective motor behaviors.
Interestingly, the stimulation frequencies that were found more effective for standing were similar to those that usually elicit rhythmic motor patterns in the supine position when the stimulation amplitude is set well above motor threshold. A similar rhythmic motor pattern can be achieved during standing, with consequent impairment of standing behavior. Thus the selection of below- or near-motor-threshold stimulation amplitudes is critical for promoting standing when applying higher stimulation frequencies. Also, lower stimulation amplitudes seem to recruit predominantly lower-threshold afferent structures, while with higher amplitudes more efferent volleys are involved, precluding the response that may have been driven by the afferent pathways ( ). This interpretation of neurophysiological recordings in the supine position further supports the use of lower stimulation amplitudes when the stimulation is aimed at altering the excitability of the spinal circuitry, allowing the load-bearing-related sensory information to control the circuitry for generating extensor motor patterns. It is also worth noting that the higher-frequency and lower-amplitude stimulation approach has been found effective for promoting standing in complete spinal rats ( ).
Role of Activity-Based Training With Spinal Cord Epidural Stimulation for the Recovery of Motor Function for Standing
Activity-based training, with or without scES, has been recognized as one of the key factors for the recovery of motor function after complete spinal cord injury in animal models by promoting training-induced neural plasticity. Recent findings also pointed out that task-specific stand training with scES parameters optimized for standing played a major role in the recovery of standing ability in chronic complete spinal cord injury individuals. The progressive improvement in standing ability was associated with a less variable EMG pattern, and with neurophysiological adaptations that conceivably reflected the strengthening of sensorimotor pathways and interneuronal connections responsible for the promotion of the continuous extension patterns that are required for standing. Interestingly, the positive effects of activity-based training on standing behavior seem to be task specific, as the subsequent step training with epidural stimulation impaired the ability to stand in three out of four individuals. This finding can be explained by the fact that standing and stepping differ considerably in terms of neural activation patterns and afferent information received by the spinal circuitry. This is relevant given previous findings which showed that training in a specific motor task can impair the spinal cord ability to perform a different motor activity in complete spinal mammals, and encourages additional scientific efforts to study different activity-based rehabilitation protocols with scES (e.g., interleaving stand and step training) that may promote the concurrent improvement of motor patterns effective for both standing and stepping.
After a severe spinal cord injury, postural control during standing or walking is also compromised. Motor-complete spinal cord injury individuals did not show any recovery of balance control during standing with scES; in fact, they needed balance assistance to maintain upright posture both before and after stand and step training ( ). However, significant motor-pattern modulation was detected during standing in response to controlled lateral and sagittal bodyweight shifting ( ; unpublished observations). These observations support the view that further studies are needed to understand the extent of postural control recovery that can be brought about by the abovementioned interventions following severe spinal cord injury in both animal models and humans.
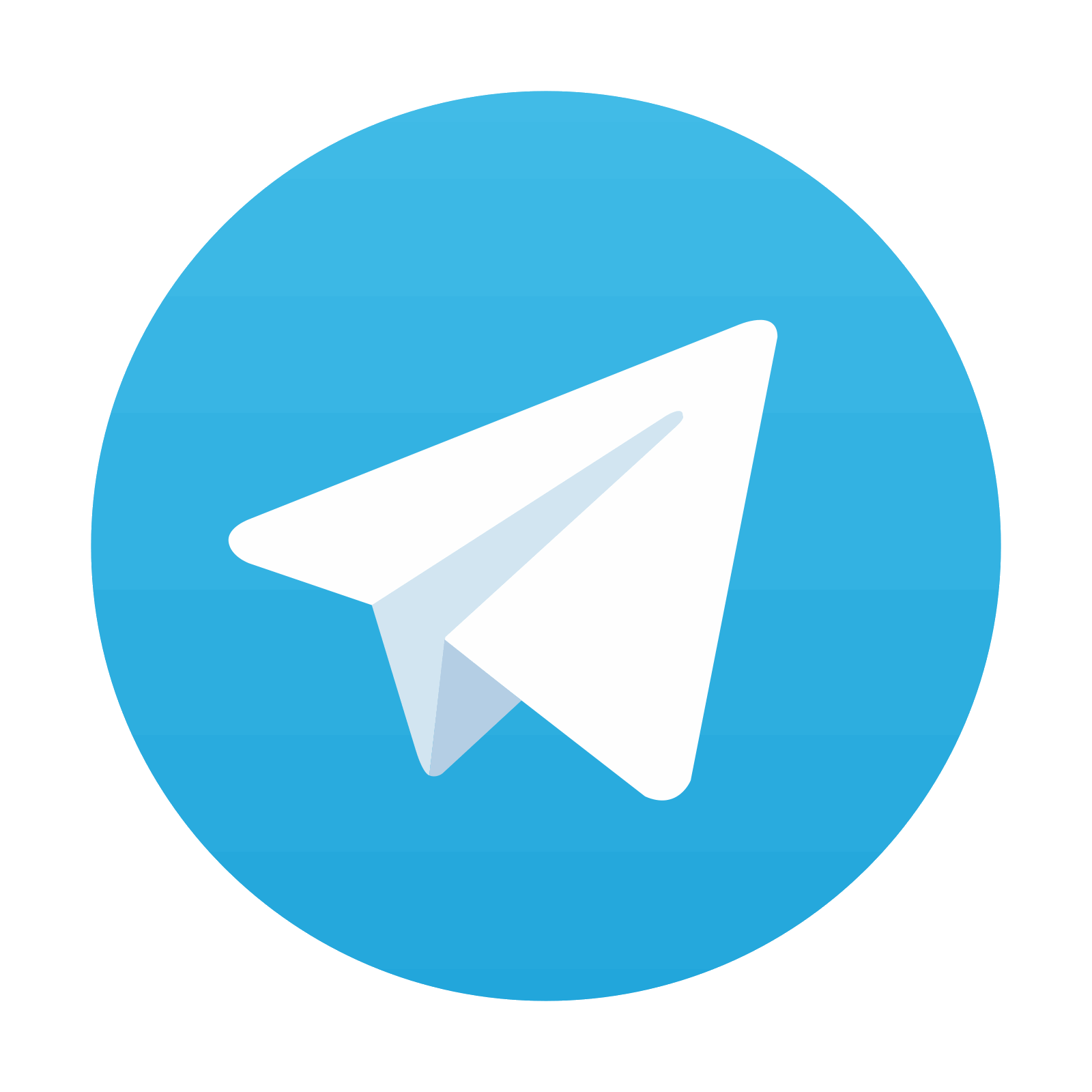
Stay updated, free articles. Join our Telegram channel
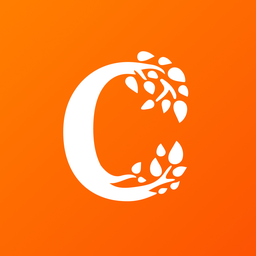
Full access? Get Clinical Tree
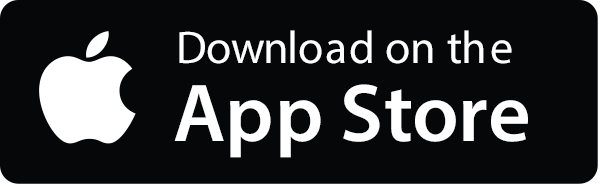
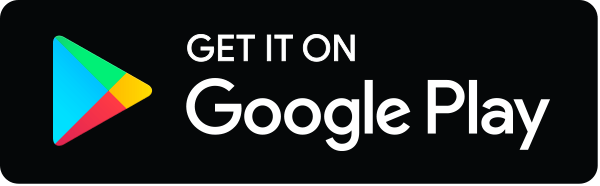