Abstract
When an individual loses a limb, they lose touch with the world and people around them. Somatosensation is critical to the feeling of connection and control of one’s own body. Loss of somatosensation may be even more devastating than loss of hand function. Advanced anthropomorphic mechatronics in sophisticated prostheses will require somatosensory feedback to operate the devices in more than a rudimentary, preprogrammed set of motions. Without sensory connection to the prosthesis it remains only an adaptive tool, not a replacement for the hand. Replacing somatosensory perception requires complex restoration of many different, coordinated signals. The peripheral nervous system is a common pathway of these signals. This chapter describes neuroprostheses for direct stimulation of peripheral nerves with appropriate patterns of information can restore the perception of somatosensation.
Keywords
Amputee, Feedback, Flat interface nerve electrode (FINE), Limb loss, Longitudinal intrafascicular electrode (LIFE), Neural interface, Neuroprosthesis, Patterned stimulation, Prosthesis, Sensation, Somatosensation, Transverse intrafascicular multichannel electrode (TIME), Upper limb
Outline
Introduction 1249
Somatosensation for Prostheses 1250
Neural Interfaces Are Needed to Restore Somatosensation 1251
Peripheral Nerve Interfaces 1252
Stimulation Parameters and Patterns 1252
Class III Medical Device Status 1253
Clinical Demonstrations of Somatosenstory Restoration 1254
Summary 1256
References 1257
Introduction
One of the most beautiful words is touch; it exquisitely represents our need to prove ourselves that we are not in a dream!
Feelings aroused by the touch of someone’s hand… work on both the unconscious and the conscious aspects of the self, and they have physiological consequences, as well.
When an individual loses a limb, they lose touch with the world and people around them. Somatosensation is critical to the feeling of connection and control of one’s own body. Loss of somatosensation may be even more detrimental than loss of hand function. Advanced anthropomorphic mechatronics in sophisticated prostheses will require somatosensory feedback to operate the devices in more than a rudimentary, preprogrammed set of motions. Without sensory connection to the prosthesis it remains only an adaptive tool, not a replacement for the hand. Rejection and abandonment of prostheses ranges from 40% to 60% of limb loss individuals ( ) with sensory feedback reported as a key factor for abandonment ( ) and a consumer design priority ( ).
Somatosensation ( ) arises from a complex system of biological organs that respond to external inputs and body state. The sensors include rapidly and slowly adapting mechanoreceptors, temperature, stretch, pain, tendon tension, muscle bag and chain fibers, and joint sensors. These receptors are found in the skin, muscles, tendons, joints, epithelial tissues, bones, organs, and cardiovascular system. Somatosensation is ubiquitous. For those without sensory loss, the normal experiences that might come close to it include the numbness following prolonged skin exposure to cold temperatures; local anesthetics for a dental procedure; or upon waking after occluding blood flow to the arm while sleeping, resulting in a “dead limb” in the morning. Replacing somatosensory perception requires complex restoration of many different, coordinated signals. The peripheral nervous system is a common pathway of these signals. Direct stimulation of peripheral nerves with appropriate patterns of information can restore the perception of somatosensation.
Somatosensation for Prostheses
Benefits
Embodiment or incorporation is the experience of incorporating the prosthesis into one’s body image and actually perceive the prosthesis as part of themselves ( ). Without incorporation, a prosthesis is an artificial tool attached to the end of the residual limb. When embodied, the prosthesis feels either like it is their hand or that their phantom is directly in the prosthesis ( ). Embodiment provides the individual the sense of being whole again. Practically, the perception of their hand performing a task is easier to control than an attached, disembodied tool ( ). Techniques for promoting embodiment include mirror box therapy ( ), robotic therapies ( ), and task-oriented focus on use of the prosthetic rather than the prosthesis itself ( ). These techniques demonstrated improvement, but without sensation, the visual experience of the prosthesis interacting with the environment is incongruous with the perceived experience, thereby disrupting the sense of embodiment ( ). Even one’s own body will feel disembodied and foreign with the loss of sensation, such as in hemi-neglect following stroke ( ). Functional magnetic resonance imaging (fMRI) studies, show that sensation is related to restoring a sense of embodiment following limb loss ( ).
Somatosensation is necessary for arbitrary control of sophisticated and complex prostheses, especially with the additional goal of reducing, not increasing, reliance on vision. Somatosensation is important to normal motor function ( ). Study of a single degree of freedom to perform a task with able-bodied subjects shows that proprioception is necessary to improve performance even when vision is present and is more important as the task difficulty increases ( ). The importance of somatosensation and motor function is evident in other disorders ( ) such as stroke ( ) and large axon neuropathies resulting in lost somatosensation ( ). Even with a normally functioning motor system of an intact hand, patients with large axon neuropathies have difficulty controlling their otherwise intact limb. This results in high cognitive load and poor performance. Even the cortical representations of motor commands are degraded with the lack of somatosensory feedback ( ). Therefore, it is not very likely that complex prostheses with highly anthropomorphic mechatronics will function well without somatosensation. Given that increasing task complexity has increasing reliance on somatosensation, the significant investment in mechatronics would paradoxically provide less value and function to the user as the added degrees of freedom become exceedingly difficult for the user to control. Even the most complex hands will essentially be preprogrammed, patterned movement.
The psychological impact of limb loss is debilitating. Depression and posttraumatic stress are common following traumatic limb loss ( ), correlated with long-term psychosocial adjustment ( ), and more prevalent following upper extremity amputation than lower extremity amputation ( ). Psychotherapy is recommended care following limb loss ( ). Depression can lead to self-destructive behavior ( ), disengagement from society ( ), lost productivity ( ), pharmacological dependence ( ), and stress on family units ( ). Depending on the cultural background, limb loss makes one feel defective or less of a human. The lack of tactile interaction with a family member results in a feeling of disconnection and lack of intimacy ( ). Use of a prosthesis provides a sense of normalcy and improvement in psychosocial interaction as users reported a reduced awareness of being different ( ). Since restoring sensation is expected to increase embodiment and connection, it is expected to further alleviate psychosocial effects of traumatic limb loss.
Pain is often associated with limb loss ( ). The physical and neurological cause of pain is complex and an active area of research. There is evidence that underlying dysfunction of somatosensation is related to the chronic pain of complex regional pain syndrome ( ). Pain, however, is a complex phenomenon with many interrelated causes that can range from being neurogenic from activity of pain fibers within a sensitive neuroma and other peripheral activity ( ), to changes in central connectivity resulting from loss of sensory input ( ) ( Fig. 103.1 ). Pain can result in reduced quality of life ( ) including loss of productivity, further deepening depression, and additional medication costs with associated risks of dependency ( ).

Body-Powered Versus Electrically Powered Prostheses and Somatosensation
Body-powered prostheses are often preferred over more functional electrical devices because the user can “feel” the grip interaction with objects via referred sensation through the body harness operating the prosthesis ( ). The representative sensory information does not directly match the user’s visual experience of the prosthesis, but does provide a sense of control and connection to the device. Despite the negative aspects of body-powered prostheses, including being limited to one active degree of freedom ( ), limited range of motion ( ) (e.g., reaching over shoulder can be nonfunctional), and cumbersome and uncomfortable nature of the body harness, many users still prefer the body-powered prosthesis to the powered devices for the referred sensation ( ). Powered prostheses have many functional advantages to body-powered devices, but unfortunately, the only somatosensory feedback is the forces applied to the residual limb through the socket, the audible feedback of the motors, and visual feedback. Prosthetic choice is often a choice between either function or sensation ( ).
Neural Interfaces Are Needed to Restore Somatosensation
Several sensory substitution techniques not requiring implanted components have been developed ( ). These include sensory substitution with cutaneous electrical stimulation ( ), vibration on the skin surface ( ), skin stretch ( ), air-bladders ( ), tendon vibration for proprioception ( ), and targeted sensory reinnervation ( ). Substitution systems introduce tactile information that is out of the normal sensorimotor pathways. Hence, interpretation of the sensation requires a conscious level of attention, and hence, has an associated cognitive load. In lab settings, these have been successful, but none have been sufficiently robust or effective in everyday use for widespread adoption in commercially available prosthetics.
When the limb is lost, the sensory end organs are lost but the nerves and pathways connecting those organs to the cerebellar, thalamic relays, and brain are available and functioning ( ). Artificial activation of these pathways results in the perception of sensation as though it was from the missing limb, hence taking advantage of both the conscious and subconscious processing and integration of sensorimotor information. There are several locations to interact with the residual somatosensory system ( ). Preclinical work in brain ( ), dorsal root ganglion ( ), and intraspinal microstimulation ( ) approaches are progressing. Intraoperative stimulation of the thalamus shows sensory percepts with electrical stimulation ( ). Peripheral interfaces have achieved chronic clinical demonstration for restoring sensation ( ) and central stimulation of the sensory cortex has shown chronic restoration of sensation for an individual with spinal cord injury ( ).
Generally, the peripheral nerve approach is a better balance of the risk-benefit ratio for those with limb loss, while cortical stimulation is required for high-level spinal cord injury. Focusing toward amputees, there are several options for peripheral nerve interfaces ( ). Most often, peripheral interfaces focus on the physical interaction and location of electrical contacts. Though infrequently discussed, the electromagnetic interaction with the nerve is equally important to the functional result of somatosensory stimulation.
Peripheral Nerve Interfaces
Generally, peripheral nerve interfaces can be divided into extraneural and intraneural categories. Extraneural electrodes surround a peripheral nerve, but do not insert elements within the nerve, causing the least disturbance to the neural tissue. Circular, self-sizing electrodes, such as the Spiral ( ) and Helical electrode ( ), have been stable in clinical applications for decades ( ). A circular configuration has the minimum surface area to interact with the neural tissue. To increase the surface area and proximity to more of the neural tissue while not penetrating the nerve, another class of extraneural electrodes maintain an elongated or rectangular configuration for the nerve. The Flat Interface Nerve Electrode (FINE) ( ) has shown fascicular level selectivity for stimulation ( ) and recording capabilities ( ). The spiral and FINE electrodes have been in clinical trials with limb loss subjects for over three years ( ).
Intraneural interfaces physically insert electrical contacts within the nerve. The least invasive designs penetrate the epineurium, but not the perineurium. Two examples include the Slowly Penetrating Interfascicular Nerve Electrode (SPINE) ( ) and the grove electrode ( ). The most invasive penetrates perineurium to place components inside the fascicle. Examples include the Longitudinal Intrafascicular Electrode (LIFE) ( ), Transverse Intrafascicular Multichannel Electrode (TIME) ( ), and Utah Slanted Electrode Array (USEA) ( ).
The perineurium is a living cellular membrane that helps maintain a blood-nerve-barrier within the fascicle and maintains the chemical balances with the fascicle for proper nerve function ( ). The objective of increasing intimacy is a one-to-one relationship between contacts and axons. There is a stochastic distribution of Nodes of Ranvier and axon sizes that results in a distributed recruitment within the nerve limiting the maximum effective electrode density ( ). When placing electrodes within the nerve, there are spatial constraints that limit the numbers of electrodes within the constrained fascicle ( ), especially when considering inflammatory response characteristics ( ), which will further reduce the intimacy of contacts with axons. Prior short-term applications of intrafascicular approaches showed increasing stimulation thresholds ( ). The number of reported sensory locations and perceptions elicited was similar to long-term extraneural approaches ( ). The functional performance of an intrafascicular approach is similar to the extraneural approach ( ).
Stimulation Parameters and Patterns
In addition to physical proximity, a second powerful tool that is often not considered is the manipulation and design of the spatiotemporal electromagnetic field. Multiple electrical contacts in electrodes like the FINE ( ) allow for the summation of electrical fields to shape and move areas of activation within the nerve. Therefore, the sensory locations are not limited to the number of electrical contacts. It has been demonstrated clinically that additional sensory perceptions are possible ( ) and the number of additional sensations possible is an active area of research.
Pulse shapes . The magnitude of the electrical fields decay by a factor of 1/ r , where r is the distance from the electrode. Nerve activation is proportional to the rate of change of the voltage difference along the axon ( ). Hence, with simple square pulses, closer, larger, myelinated axons will be activated before smaller, distant, and unmyelinated axons. However, axonal excitation is also controlled by the nonlinear dynamics of the membrane channels ( ) that can be manipulated by changing the shape of the pulse. Types of waveforms that have been explored include quasitrapezoidal pulses designed to recruit small axons before the large ( ) and ramp pulses to activate more distal axons before closer axons ( ). To minimize the power requirements for implanted devices, exponential-shaped waveforms activate the larger and closer axons in the same order, but with minimized energy ( ).
Frequency modulation . Axons convey information by spikes across a population of axons. In any single axon, the information is carried by the spike rate or frequency. Bursting pulse trains activating a small population of neurons can change the quality of tactile perceptions ( ). Changing stimulation frequency in somatosensory applications is related to intensity ( ). In studies with intact hands, increasing the intensity of either mechanical pressure or vibration results in (1) an increase in the firing rate of nerve fibers with receptive fields in the center of the mechanic stimuli and (2) the recruitment of fibers with nearby receptive fields ( ). With electrical stimulation, it is possible to separately vary stimulation frequency and number of fibers recruited. Using this model, it is shown that intensity is encoded in the total spike rate ( ). The total spike rate is related to the magnitude of the stimulation strength above threshold multiplied by the frequency of the stimulation. This is call the activation charge rate (ACR, Eq. 103.1 ).
ACR=fstim(Qstim−Qthr)
ACR=fstim(Qstim−Qthr)
ACR = f stim ( Q stim − Q thr )
Patterned stimulation intensity (PSI) . In populations of axons, additional information is conveyed by the relative timing and rates of spikes on different axons. In patterned frequency paradigms, stimulation strength is typically maintained constant for all pulses, resulting in synchronous activity in the entire axon population. This does not convey the complex information normally associated with relative differences in timing and frequency of spikes resulting from the different tactile sensors of the skin. For example, the rapidly adapting fibers burst strongly at the beginning and end of the grasp of an object while the slowly adapting fibers fire pseudocontinuously, but with a rate proportionate to the applied forces on the skin.
The generic stimulation waveform for simulation on the j th contact, Λ j , (Eq. 103.2) consists of a series of pulses that can vary on a pulse-by-pulse basis. For each pulse, the waveform shape of each pulse, ψ j,i , and the parameters of the pulse, Δ j , i , can change according to a time-depended pattern and in response to the sensory variable being measured, ϕ i . Eq. (103.2) represents the pulse from the j th electrode. The population of axons can be further modulated by adding fields from multiple channels to shape the stimulation field ( ).
Λj(ϕ,Δ,t)=∑iψj,i(Δj,i(ϕi),t−ti)ti=ti−1+IPI(ϕi−1)ϕ(t)≜Theinputormeasuredsensorydataψ≜IndividualstimuluspulsewaveformΔ(ϕ,t)≜Stimulationpulseparameters,whicharedependentonψ,ϕ,andt.
Λj(ϕ,Δ,t)=∑iψj,i(Δj,i(ϕi),t−ti)ti=ti−1+IPI(ϕi−1)ϕ(t)≜Theinputormeasuredsensorydataψ≜IndividualstimuluspulsewaveformΔ(ϕ,t)≜Stimulationpulseparameters,whicharedependentonψ,ϕ,andt.
Λ j ( ϕ , Δ , t ) = ∑ i ψ j , i ( Δ j , i ( ϕ i ) , t − t i ) t i = t i − 1 + I P I ( ϕ i − 1 ) ϕ ( t ) ≜ The input or measured sensory data ψ ≜ Individual stimulus pulse waveform Δ ( ϕ , t ) ≜ Stimulation pulse parameters, which are dependent on ψ , ϕ , and t .
The modulation of the stimulation strength and field is referred to as patterned stimulation intensity or PSI. The patterned shift in the field strength or shape over several stimulation pulses changes the axons activated with each pulse, and hence create a nonsynchronous activity in a population. If controlled properly, this information can be sufficient to restore several different somatosensation percepts ( ). Defining the function g (Δ) to be proportional to the number of neurons activated by the pulse and combining Eqs. (103.1) and (103.2) leads to the calculation of
IPI=kI(ϕ)g(Δ)
IPI=kI(ϕ)g(Δ)
I P I = k I ( ϕ ) g ( Δ )
Class III Medical Device Status
Implanted devices are US Food and Drug Administration (FDA) regulated, Class III devices (US regulation 21 CFR 860). This is a significant shift from the Class I designation of traditional prostheses and de novo Class II designation of newer, more complex prosthetic devices. Class III devices require significant oversight and regulation, which increases cost and complexity. This will be difficult to manage in the relatively small limb-loss market. Powered prostheses are already expensive and require extensive justification to the Centers for Medicare and Medicaid Services (CMS) and other third-party payers. It is important to show that the added cost of somatosensation is justified. Studies and quantification of pain relief, return to work, lower abandonment, and improved psychological outlook will be important to bringing these devices to the market. It will also be important to develop and leverage device technology that has applications in larger market segments.
Clinical Demonstrations of Somatosenstory Restoration
There are a growing number of studies providing evidence of the importance and capability of providing sensation through neural interfaces and electrical stimulation. The concept was first demonstrated by Frank Clippinger in 1974 with single-channel cuff electrodes placed around the median nerves for the upper extremity ( ) and the femoral nerve in the lower extremities ( ). Sensation was perceived on the phantom, but was generally diffuse sensation, unstable, and the quality of the sensation was generally described as paresthesia. Nerve stimulation for somatosensory restoration was then largely ignored until 2005 when a series of papers were published describing the acute trials with the LIFE electrodes placed in the median nerve ( ). There were localized sensory percepts with perceptual intensity proportional to stimulation frequency and a spread of sensory area with increased stimulation intensity. Even 25 years postinjury, a subject could still feel localized sensation within the phantom. The sensory changes associated with brain plasticity following limb loss, were not permanent and the cortical hand representation remain. In further acute trials a TIME ( ) also showed three points of sensation, a graded perceptual response, and basic object discrimination based on relative sensor activation ( ) ( Fig. 103.2 ). Subjects are able to discriminate pulses in sensory stimulation as they run their fingers over a ridged pattern and are able to distinguish between different grating patterns, showing integration of sensory experience with active surface exploration to determine information about the environment ( ). The USEA has shown sensory feedback in clinical trials of less than 30 days ( ), but stability is not yet demonstrated.

Stimulation of the sensory cortex can also provide sensory percepts that are identified as located on the extremity ( ). Two 2.4 mm × 4 mm microwire arrays with 60 electrodes arranged in a 6 × 10 grid were implanted in the sensory cortex of a subject with spinal cord injury. Stimulation produced tactile perceptions in the subject’s hand ( ). The locations were limited mostly to the distal aspect of the palm and proximal and medial phalanx of the index finger corresponded to the region of the somatosensory cortex where the arrays were implanted. The stimulation provided a variant of qualities and naturalness ( Fig. 103.3 ).

Extraneural electrodes have shown long-term, stable, multinerve, multichannel restoration of sensation with percutaneous systems with wires directly through an osseointegrated post ( ) ( Fig. 103.4 ) or through the skin ( ) ( Fig. 103.5 ). Subjects have been implanted for more than four years with FINEs on the median, ulnar, and radial nerves and spiral electrodes on the radial or ulnar nerve. The extraneural electrodes were able to produce highly localized sensations from individual contacts and sensations from many contacts were distributed over the entire hand. In one subject, the electrodes are implanted in the forearm and in a second subject, they are in the upper arm. More than 90% of the eight electrical contacts around each nerve produced somatosensation at unique locations. There is a maintained somatotopic organization of somatosensation from the hand to at least the middle upper arm. These locations and stimulation thresholds have been stable for the entire duration of the implant. Adding fields from multiple electrodes produce sensation at additional locations on the hand. The stimulation thresholds and electrode impedances were stable for the reported two-year pulse duration of the implant and are still ongoing at the time of the writing of this chapter ( ).


Different PSIs result in the subject reporting several different qualities of sensation at a single location on the hand ( ). Several of these sensations were described by the subject as being very “natural.” Different stimulation patterns resulted in sensations such as constant pressure, pulsing pressure, buzzing, tapping, flutter, sandpaper, and motion. The just-noticeable-difference and discriminability of changes in stimulation ACR demonstrate a sensitivity to changes in artificial tactile intensity that is similar to that of the intact hand ( ).
Functionally, sensory feedback improves the user’s fine control of a traditional myoelectric device ( ). The user could perform delicate tasks without visual or audio feedback, relying entirely on stimulated somatosensation. These tasks were often difficult or not possible even with visual and auditory feedback.
Chronic phantom pain that had not been resolved with medication or other desensitization therapy was reduced immediately and virtually eliminated within six months of the start of the somatosensory stimulation trial ( ). Stimulation was applied only in the lab, which was every two weeks for about 6 h/session. Pain relief, however, persisted even without stimulation.
Somatosensation for Prostheses
Benefits
Embodiment or incorporation is the experience of incorporating the prosthesis into one’s body image and actually perceive the prosthesis as part of themselves ( ). Without incorporation, a prosthesis is an artificial tool attached to the end of the residual limb. When embodied, the prosthesis feels either like it is their hand or that their phantom is directly in the prosthesis ( ). Embodiment provides the individual the sense of being whole again. Practically, the perception of their hand performing a task is easier to control than an attached, disembodied tool ( ). Techniques for promoting embodiment include mirror box therapy ( ), robotic therapies ( ), and task-oriented focus on use of the prosthetic rather than the prosthesis itself ( ). These techniques demonstrated improvement, but without sensation, the visual experience of the prosthesis interacting with the environment is incongruous with the perceived experience, thereby disrupting the sense of embodiment ( ). Even one’s own body will feel disembodied and foreign with the loss of sensation, such as in hemi-neglect following stroke ( ). Functional magnetic resonance imaging (fMRI) studies, show that sensation is related to restoring a sense of embodiment following limb loss ( ).
Somatosensation is necessary for arbitrary control of sophisticated and complex prostheses, especially with the additional goal of reducing, not increasing, reliance on vision. Somatosensation is important to normal motor function ( ). Study of a single degree of freedom to perform a task with able-bodied subjects shows that proprioception is necessary to improve performance even when vision is present and is more important as the task difficulty increases ( ). The importance of somatosensation and motor function is evident in other disorders ( ) such as stroke ( ) and large axon neuropathies resulting in lost somatosensation ( ). Even with a normally functioning motor system of an intact hand, patients with large axon neuropathies have difficulty controlling their otherwise intact limb. This results in high cognitive load and poor performance. Even the cortical representations of motor commands are degraded with the lack of somatosensory feedback ( ). Therefore, it is not very likely that complex prostheses with highly anthropomorphic mechatronics will function well without somatosensation. Given that increasing task complexity has increasing reliance on somatosensation, the significant investment in mechatronics would paradoxically provide less value and function to the user as the added degrees of freedom become exceedingly difficult for the user to control. Even the most complex hands will essentially be preprogrammed, patterned movement.
The psychological impact of limb loss is debilitating. Depression and posttraumatic stress are common following traumatic limb loss ( ), correlated with long-term psychosocial adjustment ( ), and more prevalent following upper extremity amputation than lower extremity amputation ( ). Psychotherapy is recommended care following limb loss ( ). Depression can lead to self-destructive behavior ( ), disengagement from society ( ), lost productivity ( ), pharmacological dependence ( ), and stress on family units ( ). Depending on the cultural background, limb loss makes one feel defective or less of a human. The lack of tactile interaction with a family member results in a feeling of disconnection and lack of intimacy ( ). Use of a prosthesis provides a sense of normalcy and improvement in psychosocial interaction as users reported a reduced awareness of being different ( ). Since restoring sensation is expected to increase embodiment and connection, it is expected to further alleviate psychosocial effects of traumatic limb loss.
Pain is often associated with limb loss ( ). The physical and neurological cause of pain is complex and an active area of research. There is evidence that underlying dysfunction of somatosensation is related to the chronic pain of complex regional pain syndrome ( ). Pain, however, is a complex phenomenon with many interrelated causes that can range from being neurogenic from activity of pain fibers within a sensitive neuroma and other peripheral activity ( ), to changes in central connectivity resulting from loss of sensory input ( ) ( Fig. 103.1 ). Pain can result in reduced quality of life ( ) including loss of productivity, further deepening depression, and additional medication costs with associated risks of dependency ( ).

Body-Powered Versus Electrically Powered Prostheses and Somatosensation
Body-powered prostheses are often preferred over more functional electrical devices because the user can “feel” the grip interaction with objects via referred sensation through the body harness operating the prosthesis ( ). The representative sensory information does not directly match the user’s visual experience of the prosthesis, but does provide a sense of control and connection to the device. Despite the negative aspects of body-powered prostheses, including being limited to one active degree of freedom ( ), limited range of motion ( ) (e.g., reaching over shoulder can be nonfunctional), and cumbersome and uncomfortable nature of the body harness, many users still prefer the body-powered prosthesis to the powered devices for the referred sensation ( ). Powered prostheses have many functional advantages to body-powered devices, but unfortunately, the only somatosensory feedback is the forces applied to the residual limb through the socket, the audible feedback of the motors, and visual feedback. Prosthetic choice is often a choice between either function or sensation ( ).
Neural Interfaces Are Needed to Restore Somatosensation
Several sensory substitution techniques not requiring implanted components have been developed ( ). These include sensory substitution with cutaneous electrical stimulation ( ), vibration on the skin surface ( ), skin stretch ( ), air-bladders ( ), tendon vibration for proprioception ( ), and targeted sensory reinnervation ( ). Substitution systems introduce tactile information that is out of the normal sensorimotor pathways. Hence, interpretation of the sensation requires a conscious level of attention, and hence, has an associated cognitive load. In lab settings, these have been successful, but none have been sufficiently robust or effective in everyday use for widespread adoption in commercially available prosthetics.
When the limb is lost, the sensory end organs are lost but the nerves and pathways connecting those organs to the cerebellar, thalamic relays, and brain are available and functioning ( ). Artificial activation of these pathways results in the perception of sensation as though it was from the missing limb, hence taking advantage of both the conscious and subconscious processing and integration of sensorimotor information. There are several locations to interact with the residual somatosensory system ( ). Preclinical work in brain ( ), dorsal root ganglion ( ), and intraspinal microstimulation ( ) approaches are progressing. Intraoperative stimulation of the thalamus shows sensory percepts with electrical stimulation ( ). Peripheral interfaces have achieved chronic clinical demonstration for restoring sensation ( ) and central stimulation of the sensory cortex has shown chronic restoration of sensation for an individual with spinal cord injury ( ).
Generally, the peripheral nerve approach is a better balance of the risk-benefit ratio for those with limb loss, while cortical stimulation is required for high-level spinal cord injury. Focusing toward amputees, there are several options for peripheral nerve interfaces ( ). Most often, peripheral interfaces focus on the physical interaction and location of electrical contacts. Though infrequently discussed, the electromagnetic interaction with the nerve is equally important to the functional result of somatosensory stimulation.
Peripheral Nerve Interfaces
Generally, peripheral nerve interfaces can be divided into extraneural and intraneural categories. Extraneural electrodes surround a peripheral nerve, but do not insert elements within the nerve, causing the least disturbance to the neural tissue. Circular, self-sizing electrodes, such as the Spiral ( ) and Helical electrode ( ), have been stable in clinical applications for decades ( ). A circular configuration has the minimum surface area to interact with the neural tissue. To increase the surface area and proximity to more of the neural tissue while not penetrating the nerve, another class of extraneural electrodes maintain an elongated or rectangular configuration for the nerve. The Flat Interface Nerve Electrode (FINE) ( ) has shown fascicular level selectivity for stimulation ( ) and recording capabilities ( ). The spiral and FINE electrodes have been in clinical trials with limb loss subjects for over three years ( ).
Intraneural interfaces physically insert electrical contacts within the nerve. The least invasive designs penetrate the epineurium, but not the perineurium. Two examples include the Slowly Penetrating Interfascicular Nerve Electrode (SPINE) ( ) and the grove electrode ( ). The most invasive penetrates perineurium to place components inside the fascicle. Examples include the Longitudinal Intrafascicular Electrode (LIFE) ( ), Transverse Intrafascicular Multichannel Electrode (TIME) ( ), and Utah Slanted Electrode Array (USEA) ( ).
The perineurium is a living cellular membrane that helps maintain a blood-nerve-barrier within the fascicle and maintains the chemical balances with the fascicle for proper nerve function ( ). The objective of increasing intimacy is a one-to-one relationship between contacts and axons. There is a stochastic distribution of Nodes of Ranvier and axon sizes that results in a distributed recruitment within the nerve limiting the maximum effective electrode density ( ). When placing electrodes within the nerve, there are spatial constraints that limit the numbers of electrodes within the constrained fascicle ( ), especially when considering inflammatory response characteristics ( ), which will further reduce the intimacy of contacts with axons. Prior short-term applications of intrafascicular approaches showed increasing stimulation thresholds ( ). The number of reported sensory locations and perceptions elicited was similar to long-term extraneural approaches ( ). The functional performance of an intrafascicular approach is similar to the extraneural approach ( ).
Stimulation Parameters and Patterns
In addition to physical proximity, a second powerful tool that is often not considered is the manipulation and design of the spatiotemporal electromagnetic field. Multiple electrical contacts in electrodes like the FINE ( ) allow for the summation of electrical fields to shape and move areas of activation within the nerve. Therefore, the sensory locations are not limited to the number of electrical contacts. It has been demonstrated clinically that additional sensory perceptions are possible ( ) and the number of additional sensations possible is an active area of research.
Pulse shapes . The magnitude of the electrical fields decay by a factor of 1/ r , where r is the distance from the electrode. Nerve activation is proportional to the rate of change of the voltage difference along the axon ( ). Hence, with simple square pulses, closer, larger, myelinated axons will be activated before smaller, distant, and unmyelinated axons. However, axonal excitation is also controlled by the nonlinear dynamics of the membrane channels ( ) that can be manipulated by changing the shape of the pulse. Types of waveforms that have been explored include quasitrapezoidal pulses designed to recruit small axons before the large ( ) and ramp pulses to activate more distal axons before closer axons ( ). To minimize the power requirements for implanted devices, exponential-shaped waveforms activate the larger and closer axons in the same order, but with minimized energy ( ).
Frequency modulation . Axons convey information by spikes across a population of axons. In any single axon, the information is carried by the spike rate or frequency. Bursting pulse trains activating a small population of neurons can change the quality of tactile perceptions ( ). Changing stimulation frequency in somatosensory applications is related to intensity ( ). In studies with intact hands, increasing the intensity of either mechanical pressure or vibration results in (1) an increase in the firing rate of nerve fibers with receptive fields in the center of the mechanic stimuli and (2) the recruitment of fibers with nearby receptive fields ( ). With electrical stimulation, it is possible to separately vary stimulation frequency and number of fibers recruited. Using this model, it is shown that intensity is encoded in the total spike rate ( ). The total spike rate is related to the magnitude of the stimulation strength above threshold multiplied by the frequency of the stimulation. This is call the activation charge rate (ACR, Eq. 103.1 ).
ACR=fstim(Qstim−Qthr)
ACR=fstim(Qstim−Qthr)
ACR = f stim ( Q stim − Q thr )
Patterned stimulation intensity (PSI) . In populations of axons, additional information is conveyed by the relative timing and rates of spikes on different axons. In patterned frequency paradigms, stimulation strength is typically maintained constant for all pulses, resulting in synchronous activity in the entire axon population. This does not convey the complex information normally associated with relative differences in timing and frequency of spikes resulting from the different tactile sensors of the skin. For example, the rapidly adapting fibers burst strongly at the beginning and end of the grasp of an object while the slowly adapting fibers fire pseudocontinuously, but with a rate proportionate to the applied forces on the skin.
The generic stimulation waveform for simulation on the j th contact, Λ j , (Eq. 103.2) consists of a series of pulses that can vary on a pulse-by-pulse basis. For each pulse, the waveform shape of each pulse, ψ j,i , and the parameters of the pulse, Δ j , i , can change according to a time-depended pattern and in response to the sensory variable being measured, ϕ i . Eq. (103.2) represents the pulse from the j th electrode. The population of axons can be further modulated by adding fields from multiple channels to shape the stimulation field ( ).
Λj(ϕ,Δ,t)=∑iψj,i(Δj,i(ϕi),t−ti)ti=ti−1+IPI(ϕi−1)ϕ(t)≜Theinputormeasuredsensorydataψ≜IndividualstimuluspulsewaveformΔ(ϕ,t)≜Stimulationpulseparameters,whicharedependentonψ,ϕ,andt.
Λj(ϕ,Δ,t)=∑iψj,i(Δj,i(ϕi),t−ti)ti=ti−1+IPI(ϕi−1)ϕ(t)≜Theinputormeasuredsensorydataψ≜IndividualstimuluspulsewaveformΔ(ϕ,t)≜Stimulationpulseparameters,whicharedependentonψ,ϕ,andt.
Λ j ( ϕ , Δ , t ) = ∑ i ψ j , i ( Δ j , i ( ϕ i ) , t − t i ) t i = t i − 1 + I P I ( ϕ i − 1 ) ϕ ( t ) ≜ The input or measured sensory data ψ ≜ Individual stimulus pulse waveform Δ ( ϕ , t ) ≜ Stimulation pulse parameters, which are dependent on ψ , ϕ , and t .
The modulation of the stimulation strength and field is referred to as patterned stimulation intensity or PSI. The patterned shift in the field strength or shape over several stimulation pulses changes the axons activated with each pulse, and hence create a nonsynchronous activity in a population. If controlled properly, this information can be sufficient to restore several different somatosensation percepts ( ). Defining the function g (Δ) to be proportional to the number of neurons activated by the pulse and combining Eqs. (103.1) and (103.2) leads to the calculation of
IPI=kI(ϕ)g(Δ)
IPI=kI(ϕ)g(Δ)
I P I = k I ( ϕ ) g ( Δ )
Class III Medical Device Status
Implanted devices are US Food and Drug Administration (FDA) regulated, Class III devices (US regulation 21 CFR 860). This is a significant shift from the Class I designation of traditional prostheses and de novo Class II designation of newer, more complex prosthetic devices. Class III devices require significant oversight and regulation, which increases cost and complexity. This will be difficult to manage in the relatively small limb-loss market. Powered prostheses are already expensive and require extensive justification to the Centers for Medicare and Medicaid Services (CMS) and other third-party payers. It is important to show that the added cost of somatosensation is justified. Studies and quantification of pain relief, return to work, lower abandonment, and improved psychological outlook will be important to bringing these devices to the market. It will also be important to develop and leverage device technology that has applications in larger market segments.
Clinical Demonstrations of Somatosenstory Restoration
There are a growing number of studies providing evidence of the importance and capability of providing sensation through neural interfaces and electrical stimulation. The concept was first demonstrated by Frank Clippinger in 1974 with single-channel cuff electrodes placed around the median nerves for the upper extremity ( ) and the femoral nerve in the lower extremities ( ). Sensation was perceived on the phantom, but was generally diffuse sensation, unstable, and the quality of the sensation was generally described as paresthesia. Nerve stimulation for somatosensory restoration was then largely ignored until 2005 when a series of papers were published describing the acute trials with the LIFE electrodes placed in the median nerve ( ). There were localized sensory percepts with perceptual intensity proportional to stimulation frequency and a spread of sensory area with increased stimulation intensity. Even 25 years postinjury, a subject could still feel localized sensation within the phantom. The sensory changes associated with brain plasticity following limb loss, were not permanent and the cortical hand representation remain. In further acute trials a TIME ( ) also showed three points of sensation, a graded perceptual response, and basic object discrimination based on relative sensor activation ( ) ( Fig. 103.2 ). Subjects are able to discriminate pulses in sensory stimulation as they run their fingers over a ridged pattern and are able to distinguish between different grating patterns, showing integration of sensory experience with active surface exploration to determine information about the environment ( ). The USEA has shown sensory feedback in clinical trials of less than 30 days ( ), but stability is not yet demonstrated.

Stimulation of the sensory cortex can also provide sensory percepts that are identified as located on the extremity ( ). Two 2.4 mm × 4 mm microwire arrays with 60 electrodes arranged in a 6 × 10 grid were implanted in the sensory cortex of a subject with spinal cord injury. Stimulation produced tactile perceptions in the subject’s hand ( ). The locations were limited mostly to the distal aspect of the palm and proximal and medial phalanx of the index finger corresponded to the region of the somatosensory cortex where the arrays were implanted. The stimulation provided a variant of qualities and naturalness ( Fig. 103.3 ).

Extraneural electrodes have shown long-term, stable, multinerve, multichannel restoration of sensation with percutaneous systems with wires directly through an osseointegrated post ( ) ( Fig. 103.4 ) or through the skin ( ) ( Fig. 103.5 ). Subjects have been implanted for more than four years with FINEs on the median, ulnar, and radial nerves and spiral electrodes on the radial or ulnar nerve. The extraneural electrodes were able to produce highly localized sensations from individual contacts and sensations from many contacts were distributed over the entire hand. In one subject, the electrodes are implanted in the forearm and in a second subject, they are in the upper arm. More than 90% of the eight electrical contacts around each nerve produced somatosensation at unique locations. There is a maintained somatotopic organization of somatosensation from the hand to at least the middle upper arm. These locations and stimulation thresholds have been stable for the entire duration of the implant. Adding fields from multiple electrodes produce sensation at additional locations on the hand. The stimulation thresholds and electrode impedances were stable for the reported two-year pulse duration of the implant and are still ongoing at the time of the writing of this chapter ( ).


Different PSIs result in the subject reporting several different qualities of sensation at a single location on the hand ( ). Several of these sensations were described by the subject as being very “natural.” Different stimulation patterns resulted in sensations such as constant pressure, pulsing pressure, buzzing, tapping, flutter, sandpaper, and motion. The just-noticeable-difference and discriminability of changes in stimulation ACR demonstrate a sensitivity to changes in artificial tactile intensity that is similar to that of the intact hand ( ).
Functionally, sensory feedback improves the user’s fine control of a traditional myoelectric device ( ). The user could perform delicate tasks without visual or audio feedback, relying entirely on stimulated somatosensation. These tasks were often difficult or not possible even with visual and auditory feedback.
Chronic phantom pain that had not been resolved with medication or other desensitization therapy was reduced immediately and virtually eliminated within six months of the start of the somatosensory stimulation trial ( ). Stimulation was applied only in the lab, which was every two weeks for about 6 h/session. Pain relief, however, persisted even without stimulation.
Somatosensation for Prostheses
Benefits
Embodiment or incorporation is the experience of incorporating the prosthesis into one’s body image and actually perceive the prosthesis as part of themselves ( ). Without incorporation, a prosthesis is an artificial tool attached to the end of the residual limb. When embodied, the prosthesis feels either like it is their hand or that their phantom is directly in the prosthesis ( ). Embodiment provides the individual the sense of being whole again. Practically, the perception of their hand performing a task is easier to control than an attached, disembodied tool ( ). Techniques for promoting embodiment include mirror box therapy ( ), robotic therapies ( ), and task-oriented focus on use of the prosthetic rather than the prosthesis itself ( ). These techniques demonstrated improvement, but without sensation, the visual experience of the prosthesis interacting with the environment is incongruous with the perceived experience, thereby disrupting the sense of embodiment ( ). Even one’s own body will feel disembodied and foreign with the loss of sensation, such as in hemi-neglect following stroke ( ). Functional magnetic resonance imaging (fMRI) studies, show that sensation is related to restoring a sense of embodiment following limb loss ( ).
Somatosensation is necessary for arbitrary control of sophisticated and complex prostheses, especially with the additional goal of reducing, not increasing, reliance on vision. Somatosensation is important to normal motor function ( ). Study of a single degree of freedom to perform a task with able-bodied subjects shows that proprioception is necessary to improve performance even when vision is present and is more important as the task difficulty increases ( ). The importance of somatosensation and motor function is evident in other disorders ( ) such as stroke ( ) and large axon neuropathies resulting in lost somatosensation ( ). Even with a normally functioning motor system of an intact hand, patients with large axon neuropathies have difficulty controlling their otherwise intact limb. This results in high cognitive load and poor performance. Even the cortical representations of motor commands are degraded with the lack of somatosensory feedback ( ). Therefore, it is not very likely that complex prostheses with highly anthropomorphic mechatronics will function well without somatosensation. Given that increasing task complexity has increasing reliance on somatosensation, the significant investment in mechatronics would paradoxically provide less value and function to the user as the added degrees of freedom become exceedingly difficult for the user to control. Even the most complex hands will essentially be preprogrammed, patterned movement.
The psychological impact of limb loss is debilitating. Depression and posttraumatic stress are common following traumatic limb loss ( ), correlated with long-term psychosocial adjustment ( ), and more prevalent following upper extremity amputation than lower extremity amputation ( ). Psychotherapy is recommended care following limb loss ( ). Depression can lead to self-destructive behavior ( ), disengagement from society ( ), lost productivity ( ), pharmacological dependence ( ), and stress on family units ( ). Depending on the cultural background, limb loss makes one feel defective or less of a human. The lack of tactile interaction with a family member results in a feeling of disconnection and lack of intimacy ( ). Use of a prosthesis provides a sense of normalcy and improvement in psychosocial interaction as users reported a reduced awareness of being different ( ). Since restoring sensation is expected to increase embodiment and connection, it is expected to further alleviate psychosocial effects of traumatic limb loss.
Pain is often associated with limb loss ( ). The physical and neurological cause of pain is complex and an active area of research. There is evidence that underlying dysfunction of somatosensation is related to the chronic pain of complex regional pain syndrome ( ). Pain, however, is a complex phenomenon with many interrelated causes that can range from being neurogenic from activity of pain fibers within a sensitive neuroma and other peripheral activity ( ), to changes in central connectivity resulting from loss of sensory input ( ) ( Fig. 103.1 ). Pain can result in reduced quality of life ( ) including loss of productivity, further deepening depression, and additional medication costs with associated risks of dependency ( ).

Body-Powered Versus Electrically Powered Prostheses and Somatosensation
Body-powered prostheses are often preferred over more functional electrical devices because the user can “feel” the grip interaction with objects via referred sensation through the body harness operating the prosthesis ( ). The representative sensory information does not directly match the user’s visual experience of the prosthesis, but does provide a sense of control and connection to the device. Despite the negative aspects of body-powered prostheses, including being limited to one active degree of freedom ( ), limited range of motion ( ) (e.g., reaching over shoulder can be nonfunctional), and cumbersome and uncomfortable nature of the body harness, many users still prefer the body-powered prosthesis to the powered devices for the referred sensation ( ). Powered prostheses have many functional advantages to body-powered devices, but unfortunately, the only somatosensory feedback is the forces applied to the residual limb through the socket, the audible feedback of the motors, and visual feedback. Prosthetic choice is often a choice between either function or sensation ( ).
Neural Interfaces Are Needed to Restore Somatosensation
Several sensory substitution techniques not requiring implanted components have been developed ( ). These include sensory substitution with cutaneous electrical stimulation ( ), vibration on the skin surface ( ), skin stretch ( ), air-bladders ( ), tendon vibration for proprioception ( ), and targeted sensory reinnervation ( ). Substitution systems introduce tactile information that is out of the normal sensorimotor pathways. Hence, interpretation of the sensation requires a conscious level of attention, and hence, has an associated cognitive load. In lab settings, these have been successful, but none have been sufficiently robust or effective in everyday use for widespread adoption in commercially available prosthetics.
When the limb is lost, the sensory end organs are lost but the nerves and pathways connecting those organs to the cerebellar, thalamic relays, and brain are available and functioning ( ). Artificial activation of these pathways results in the perception of sensation as though it was from the missing limb, hence taking advantage of both the conscious and subconscious processing and integration of sensorimotor information. There are several locations to interact with the residual somatosensory system ( ). Preclinical work in brain ( ), dorsal root ganglion ( ), and intraspinal microstimulation ( ) approaches are progressing. Intraoperative stimulation of the thalamus shows sensory percepts with electrical stimulation ( ). Peripheral interfaces have achieved chronic clinical demonstration for restoring sensation ( ) and central stimulation of the sensory cortex has shown chronic restoration of sensation for an individual with spinal cord injury ( ).
Generally, the peripheral nerve approach is a better balance of the risk-benefit ratio for those with limb loss, while cortical stimulation is required for high-level spinal cord injury. Focusing toward amputees, there are several options for peripheral nerve interfaces ( ). Most often, peripheral interfaces focus on the physical interaction and location of electrical contacts. Though infrequently discussed, the electromagnetic interaction with the nerve is equally important to the functional result of somatosensory stimulation.
Peripheral Nerve Interfaces
Generally, peripheral nerve interfaces can be divided into extraneural and intraneural categories. Extraneural electrodes surround a peripheral nerve, but do not insert elements within the nerve, causing the least disturbance to the neural tissue. Circular, self-sizing electrodes, such as the Spiral ( ) and Helical electrode ( ), have been stable in clinical applications for decades ( ). A circular configuration has the minimum surface area to interact with the neural tissue. To increase the surface area and proximity to more of the neural tissue while not penetrating the nerve, another class of extraneural electrodes maintain an elongated or rectangular configuration for the nerve. The Flat Interface Nerve Electrode (FINE) ( ) has shown fascicular level selectivity for stimulation ( ) and recording capabilities ( ). The spiral and FINE electrodes have been in clinical trials with limb loss subjects for over three years ( ).
Intraneural interfaces physically insert electrical contacts within the nerve. The least invasive designs penetrate the epineurium, but not the perineurium. Two examples include the Slowly Penetrating Interfascicular Nerve Electrode (SPINE) ( ) and the grove electrode ( ). The most invasive penetrates perineurium to place components inside the fascicle. Examples include the Longitudinal Intrafascicular Electrode (LIFE) ( ), Transverse Intrafascicular Multichannel Electrode (TIME) ( ), and Utah Slanted Electrode Array (USEA) ( ).
The perineurium is a living cellular membrane that helps maintain a blood-nerve-barrier within the fascicle and maintains the chemical balances with the fascicle for proper nerve function ( ). The objective of increasing intimacy is a one-to-one relationship between contacts and axons. There is a stochastic distribution of Nodes of Ranvier and axon sizes that results in a distributed recruitment within the nerve limiting the maximum effective electrode density ( ). When placing electrodes within the nerve, there are spatial constraints that limit the numbers of electrodes within the constrained fascicle ( ), especially when considering inflammatory response characteristics ( ), which will further reduce the intimacy of contacts with axons. Prior short-term applications of intrafascicular approaches showed increasing stimulation thresholds ( ). The number of reported sensory locations and perceptions elicited was similar to long-term extraneural approaches ( ). The functional performance of an intrafascicular approach is similar to the extraneural approach ( ).
Stimulation Parameters and Patterns
In addition to physical proximity, a second powerful tool that is often not considered is the manipulation and design of the spatiotemporal electromagnetic field. Multiple electrical contacts in electrodes like the FINE ( ) allow for the summation of electrical fields to shape and move areas of activation within the nerve. Therefore, the sensory locations are not limited to the number of electrical contacts. It has been demonstrated clinically that additional sensory perceptions are possible ( ) and the number of additional sensations possible is an active area of research.
Pulse shapes . The magnitude of the electrical fields decay by a factor of 1/ r , where r is the distance from the electrode. Nerve activation is proportional to the rate of change of the voltage difference along the axon ( ). Hence, with simple square pulses, closer, larger, myelinated axons will be activated before smaller, distant, and unmyelinated axons. However, axonal excitation is also controlled by the nonlinear dynamics of the membrane channels ( ) that can be manipulated by changing the shape of the pulse. Types of waveforms that have been explored include quasitrapezoidal pulses designed to recruit small axons before the large ( ) and ramp pulses to activate more distal axons before closer axons ( ). To minimize the power requirements for implanted devices, exponential-shaped waveforms activate the larger and closer axons in the same order, but with minimized energy ( ).
Frequency modulation . Axons convey information by spikes across a population of axons. In any single axon, the information is carried by the spike rate or frequency. Bursting pulse trains activating a small population of neurons can change the quality of tactile perceptions ( ). Changing stimulation frequency in somatosensory applications is related to intensity ( ). In studies with intact hands, increasing the intensity of either mechanical pressure or vibration results in (1) an increase in the firing rate of nerve fibers with receptive fields in the center of the mechanic stimuli and (2) the recruitment of fibers with nearby receptive fields ( ). With electrical stimulation, it is possible to separately vary stimulation frequency and number of fibers recruited. Using this model, it is shown that intensity is encoded in the total spike rate ( ). The total spike rate is related to the magnitude of the stimulation strength above threshold multiplied by the frequency of the stimulation. This is call the activation charge rate (ACR, Eq. 103.1 ).
ACR=fstim(Qstim−Qthr)
ACR=fstim(Qstim−Qthr)
ACR = f stim ( Q stim − Q thr )
Patterned stimulation intensity (PSI) . In populations of axons, additional information is conveyed by the relative timing and rates of spikes on different axons. In patterned frequency paradigms, stimulation strength is typically maintained constant for all pulses, resulting in synchronous activity in the entire axon population. This does not convey the complex information normally associated with relative differences in timing and frequency of spikes resulting from the different tactile sensors of the skin. For example, the rapidly adapting fibers burst strongly at the beginning and end of the grasp of an object while the slowly adapting fibers fire pseudocontinuously, but with a rate proportionate to the applied forces on the skin.
The generic stimulation waveform for simulation on the j th contact, Λ j , (Eq. 103.2) consists of a series of pulses that can vary on a pulse-by-pulse basis. For each pulse, the waveform shape of each pulse, ψ j,i , and the parameters of the pulse, Δ j , i , can change according to a time-depended pattern and in response to the sensory variable being measured, ϕ i . Eq. (103.2) represents the pulse from the j th electrode. The population of axons can be further modulated by adding fields from multiple channels to shape the stimulation field ( ).
Λj(ϕ,Δ,t)=∑iψj,i(Δj,i(ϕi),t−ti)ti=ti−1+IPI(ϕi−1)ϕ(t)≜Theinputormeasuredsensorydataψ≜IndividualstimuluspulsewaveformΔ(ϕ,t)≜Stimulationpulseparameters,whicharedependentonψ,ϕ,andt.
Λj(ϕ,Δ,t)=∑iψj,i(Δj,i(ϕi),t−ti)ti=ti−1+IPI(ϕi−1)ϕ(t)≜Theinputormeasuredsensorydataψ≜IndividualstimuluspulsewaveformΔ(ϕ,t)≜Stimulationpulseparameters,whicharedependentonψ,ϕ,andt.
Λ j ( ϕ , Δ , t ) = ∑ i ψ j , i ( Δ j , i ( ϕ i ) , t − t i ) t i = t i − 1 + I P I ( ϕ i − 1 ) ϕ ( t ) ≜ The input or measured sensory data ψ ≜ Individual stimulus pulse waveform Δ ( ϕ , t ) ≜ Stimulation pulse parameters, which are dependent on ψ , ϕ , and t .
The modulation of the stimulation strength and field is referred to as patterned stimulation intensity or PSI. The patterned shift in the field strength or shape over several stimulation pulses changes the axons activated with each pulse, and hence create a nonsynchronous activity in a population. If controlled properly, this information can be sufficient to restore several different somatosensation percepts ( ). Defining the function g (Δ) to be proportional to the number of neurons activated by the pulse and combining Eqs. (103.1) and (103.2) leads to the calculation of
IPI=kI(ϕ)g(Δ)
I P I = k I ( ϕ ) g ( Δ )
Class III Medical Device Status
Implanted devices are US Food and Drug Administration (FDA) regulated, Class III devices (US regulation 21 CFR 860). This is a significant shift from the Class I designation of traditional prostheses and de novo Class II designation of newer, more complex prosthetic devices. Class III devices require significant oversight and regulation, which increases cost and complexity. This will be difficult to manage in the relatively small limb-loss market. Powered prostheses are already expensive and require extensive justification to the Centers for Medicare and Medicaid Services (CMS) and other third-party payers. It is important to show that the added cost of somatosensation is justified. Studies and quantification of pain relief, return to work, lower abandonment, and improved psychological outlook will be important to bringing these devices to the market. It will also be important to develop and leverage device technology that has applications in larger market segments.
Clinical Demonstrations of Somatosenstory Restoration
There are a growing number of studies providing evidence of the importance and capability of providing sensation through neural interfaces and electrical stimulation. The concept was first demonstrated by Frank Clippinger in 1974 with single-channel cuff electrodes placed around the median nerves for the upper extremity ( ) and the femoral nerve in the lower extremities ( ). Sensation was perceived on the phantom, but was generally diffuse sensation, unstable, and the quality of the sensation was generally described as paresthesia. Nerve stimulation for somatosensory restoration was then largely ignored until 2005 when a series of papers were published describing the acute trials with the LIFE electrodes placed in the median nerve ( ). There were localized sensory percepts with perceptual intensity proportional to stimulation frequency and a spread of sensory area with increased stimulation intensity. Even 25 years postinjury, a subject could still feel localized sensation within the phantom. The sensory changes associated with brain plasticity following limb loss, were not permanent and the cortical hand representation remain. In further acute trials a TIME ( ) also showed three points of sensation, a graded perceptual response, and basic object discrimination based on relative sensor activation ( ) ( Fig. 103.2 ). Subjects are able to discriminate pulses in sensory stimulation as they run their fingers over a ridged pattern and are able to distinguish between different grating patterns, showing integration of sensory experience with active surface exploration to determine information about the environment ( ). The USEA has shown sensory feedback in clinical trials of less than 30 days ( ), but stability is not yet demonstrated.

Stimulation of the sensory cortex can also provide sensory percepts that are identified as located on the extremity ( ). Two 2.4 mm × 4 mm microwire arrays with 60 electrodes arranged in a 6 × 10 grid were implanted in the sensory cortex of a subject with spinal cord injury. Stimulation produced tactile perceptions in the subject’s hand ( ). The locations were limited mostly to the distal aspect of the palm and proximal and medial phalanx of the index finger corresponded to the region of the somatosensory cortex where the arrays were implanted. The stimulation provided a variant of qualities and naturalness ( Fig. 103.3 ).

Extraneural electrodes have shown long-term, stable, multinerve, multichannel restoration of sensation with percutaneous systems with wires directly through an osseointegrated post ( ) ( Fig. 103.4 ) or through the skin ( ) ( Fig. 103.5 ). Subjects have been implanted for more than four years with FINEs on the median, ulnar, and radial nerves and spiral electrodes on the radial or ulnar nerve. The extraneural electrodes were able to produce highly localized sensations from individual contacts and sensations from many contacts were distributed over the entire hand. In one subject, the electrodes are implanted in the forearm and in a second subject, they are in the upper arm. More than 90% of the eight electrical contacts around each nerve produced somatosensation at unique locations. There is a maintained somatotopic organization of somatosensation from the hand to at least the middle upper arm. These locations and stimulation thresholds have been stable for the entire duration of the implant. Adding fields from multiple electrodes produce sensation at additional locations on the hand. The stimulation thresholds and electrode impedances were stable for the reported two-year pulse duration of the implant and are still ongoing at the time of the writing of this chapter ( ).


Different PSIs result in the subject reporting several different qualities of sensation at a single location on the hand ( ). Several of these sensations were described by the subject as being very “natural.” Different stimulation patterns resulted in sensations such as constant pressure, pulsing pressure, buzzing, tapping, flutter, sandpaper, and motion. The just-noticeable-difference and discriminability of changes in stimulation ACR demonstrate a sensitivity to changes in artificial tactile intensity that is similar to that of the intact hand ( ).
Functionally, sensory feedback improves the user’s fine control of a traditional myoelectric device ( ). The user could perform delicate tasks without visual or audio feedback, relying entirely on stimulated somatosensation. These tasks were often difficult or not possible even with visual and auditory feedback.
Chronic phantom pain that had not been resolved with medication or other desensitization therapy was reduced immediately and virtually eliminated within six months of the start of the somatosensory stimulation trial ( ). Stimulation was applied only in the lab, which was every two weeks for about 6 h/session. Pain relief, however, persisted even without stimulation.
Summary
Chronic restoration of somatosensation has been shown for individuals with limb loss ( ). Neural interfaces with sophisticated stimulation paradigms create a user’s perception of their hand to touch and manipulate objects. Multichannel stimulation of the peripheral nerve can control location and size of somatosensation perception. The pattern of intensity and frequency of stimulation is critical to the quality and intensity of perceived sensation. Restoring feeling has allowed the individuals to, “feel [my] hand for the first time since the accident,” and “feel [my] wife touch my hand.” These individuals using a prosthetic hand with sensation can pull cherries and grapes from the stem, open water bottles, and move objects without destroying these objects—all while aurally and visually deprived. Phantom pain has been virtually eliminated in these two subjects. There are several technologies in development, but extraneural peripheral nerve interfaces have been stable for more than three years in two different studies ( ). Restoration of somatosensation following limb loss is now tangible.
This review has focused on upper limb loss. There is a more substantial population and need for individuals with lower limb loss. Stimulation of lower extremity peripheral nerves is expected to similarly produce sensation in the phantom foot. Sensation would be valuable to restore a sense of ground strike, foot position, and center of pressure during standing and locomotion.
In addition to the value in prosthetics, these reports drive important research questions in neurology. A few specific patterns of stimulation intensity alter the quality of the perceptual experience. It is unlikely that the patterns reproduce the exact neural activity of the intact somatosensory system. The somatosensory processing system, however, can accommodate a small amount of noise or error and recognize a pattern based on prior experience. The important questions yet to address are (1) how much noise is acceptable to still achieve accurate classification; (2) how many different perceptions can be recognized; and (3) how does learning with long-term constant application of stimulation patterns change the “naturalness” and recognition of different sensory perceptions.
Restoration of sensation through natural peripheral and central cortical pathways has achieved substantial clinical demonstration and can be considered as an important component of neuromodulation systems.
Somatosensation for Prostheses
Benefits
Embodiment or incorporation is the experience of incorporating the prosthesis into one’s body image and actually perceive the prosthesis as part of themselves ( ). Without incorporation, a prosthesis is an artificial tool attached to the end of the residual limb. When embodied, the prosthesis feels either like it is their hand or that their phantom is directly in the prosthesis ( ). Embodiment provides the individual the sense of being whole again. Practically, the perception of their hand performing a task is easier to control than an attached, disembodied tool ( ). Techniques for promoting embodiment include mirror box therapy ( ), robotic therapies ( ), and task-oriented focus on use of the prosthetic rather than the prosthesis itself ( ). These techniques demonstrated improvement, but without sensation, the visual experience of the prosthesis interacting with the environment is incongruous with the perceived experience, thereby disrupting the sense of embodiment ( ). Even one’s own body will feel disembodied and foreign with the loss of sensation, such as in hemi-neglect following stroke ( ). Functional magnetic resonance imaging (fMRI) studies, show that sensation is related to restoring a sense of embodiment following limb loss ( ).
Somatosensation is necessary for arbitrary control of sophisticated and complex prostheses, especially with the additional goal of reducing, not increasing, reliance on vision. Somatosensation is important to normal motor function ( ). Study of a single degree of freedom to perform a task with able-bodied subjects shows that proprioception is necessary to improve performance even when vision is present and is more important as the task difficulty increases ( ). The importance of somatosensation and motor function is evident in other disorders ( ) such as stroke ( ) and large axon neuropathies resulting in lost somatosensation ( ). Even with a normally functioning motor system of an intact hand, patients with large axon neuropathies have difficulty controlling their otherwise intact limb. This results in high cognitive load and poor performance. Even the cortical representations of motor commands are degraded with the lack of somatosensory feedback ( ). Therefore, it is not very likely that complex prostheses with highly anthropomorphic mechatronics will function well without somatosensation. Given that increasing task complexity has increasing reliance on somatosensation, the significant investment in mechatronics would paradoxically provide less value and function to the user as the added degrees of freedom become exceedingly difficult for the user to control. Even the most complex hands will essentially be preprogrammed, patterned movement.
The psychological impact of limb loss is debilitating. Depression and posttraumatic stress are common following traumatic limb loss ( ), correlated with long-term psychosocial adjustment ( ), and more prevalent following upper extremity amputation than lower extremity amputation ( ). Psychotherapy is recommended care following limb loss ( ). Depression can lead to self-destructive behavior ( ), disengagement from society ( ), lost productivity ( ), pharmacological dependence ( ), and stress on family units ( ). Depending on the cultural background, limb loss makes one feel defective or less of a human. The lack of tactile interaction with a family member results in a feeling of disconnection and lack of intimacy ( ). Use of a prosthesis provides a sense of normalcy and improvement in psychosocial interaction as users reported a reduced awareness of being different ( ). Since restoring sensation is expected to increase embodiment and connection, it is expected to further alleviate psychosocial effects of traumatic limb loss.
Pain is often associated with limb loss ( ). The physical and neurological cause of pain is complex and an active area of research. There is evidence that underlying dysfunction of somatosensation is related to the chronic pain of complex regional pain syndrome ( ). Pain, however, is a complex phenomenon with many interrelated causes that can range from being neurogenic from activity of pain fibers within a sensitive neuroma and other peripheral activity ( ), to changes in central connectivity resulting from loss of sensory input ( ) ( Fig. 103.1 ). Pain can result in reduced quality of life ( ) including loss of productivity, further deepening depression, and additional medication costs with associated risks of dependency ( ).

Body-Powered Versus Electrically Powered Prostheses and Somatosensation
Body-powered prostheses are often preferred over more functional electrical devices because the user can “feel” the grip interaction with objects via referred sensation through the body harness operating the prosthesis ( ). The representative sensory information does not directly match the user’s visual experience of the prosthesis, but does provide a sense of control and connection to the device. Despite the negative aspects of body-powered prostheses, including being limited to one active degree of freedom ( ), limited range of motion ( ) (e.g., reaching over shoulder can be nonfunctional), and cumbersome and uncomfortable nature of the body harness, many users still prefer the body-powered prosthesis to the powered devices for the referred sensation ( ). Powered prostheses have many functional advantages to body-powered devices, but unfortunately, the only somatosensory feedback is the forces applied to the residual limb through the socket, the audible feedback of the motors, and visual feedback. Prosthetic choice is often a choice between either function or sensation ( ).
Neural Interfaces Are Needed to Restore Somatosensation
Several sensory substitution techniques not requiring implanted components have been developed ( ). These include sensory substitution with cutaneous electrical stimulation ( ), vibration on the skin surface ( ), skin stretch ( ), air-bladders ( ), tendon vibration for proprioception ( ), and targeted sensory reinnervation ( ). Substitution systems introduce tactile information that is out of the normal sensorimotor pathways. Hence, interpretation of the sensation requires a conscious level of attention, and hence, has an associated cognitive load. In lab settings, these have been successful, but none have been sufficiently robust or effective in everyday use for widespread adoption in commercially available prosthetics.
When the limb is lost, the sensory end organs are lost but the nerves and pathways connecting those organs to the cerebellar, thalamic relays, and brain are available and functioning ( ). Artificial activation of these pathways results in the perception of sensation as though it was from the missing limb, hence taking advantage of both the conscious and subconscious processing and integration of sensorimotor information. There are several locations to interact with the residual somatosensory system ( ). Preclinical work in brain ( ), dorsal root ganglion ( ), and intraspinal microstimulation ( ) approaches are progressing. Intraoperative stimulation of the thalamus shows sensory percepts with electrical stimulation ( ). Peripheral interfaces have achieved chronic clinical demonstration for restoring sensation ( ) and central stimulation of the sensory cortex has shown chronic restoration of sensation for an individual with spinal cord injury ( ).
Generally, the peripheral nerve approach is a better balance of the risk-benefit ratio for those with limb loss, while cortical stimulation is required for high-level spinal cord injury. Focusing toward amputees, there are several options for peripheral nerve interfaces ( ). Most often, peripheral interfaces focus on the physical interaction and location of electrical contacts. Though infrequently discussed, the electromagnetic interaction with the nerve is equally important to the functional result of somatosensory stimulation.
Peripheral Nerve Interfaces
Generally, peripheral nerve interfaces can be divided into extraneural and intraneural categories. Extraneural electrodes surround a peripheral nerve, but do not insert elements within the nerve, causing the least disturbance to the neural tissue. Circular, self-sizing electrodes, such as the Spiral ( ) and Helical electrode ( ), have been stable in clinical applications for decades ( ). A circular configuration has the minimum surface area to interact with the neural tissue. To increase the surface area and proximity to more of the neural tissue while not penetrating the nerve, another class of extraneural electrodes maintain an elongated or rectangular configuration for the nerve. The Flat Interface Nerve Electrode (FINE) ( ) has shown fascicular level selectivity for stimulation ( ) and recording capabilities ( ). The spiral and FINE electrodes have been in clinical trials with limb loss subjects for over three years ( ).
Intraneural interfaces physically insert electrical contacts within the nerve. The least invasive designs penetrate the epineurium, but not the perineurium. Two examples include the Slowly Penetrating Interfascicular Nerve Electrode (SPINE) ( ) and the grove electrode ( ). The most invasive penetrates perineurium to place components inside the fascicle. Examples include the Longitudinal Intrafascicular Electrode (LIFE) ( ), Transverse Intrafascicular Multichannel Electrode (TIME) ( ), and Utah Slanted Electrode Array (USEA) ( ).
The perineurium is a living cellular membrane that helps maintain a blood-nerve-barrier within the fascicle and maintains the chemical balances with the fascicle for proper nerve function ( ). The objective of increasing intimacy is a one-to-one relationship between contacts and axons. There is a stochastic distribution of Nodes of Ranvier and axon sizes that results in a distributed recruitment within the nerve limiting the maximum effective electrode density ( ). When placing electrodes within the nerve, there are spatial constraints that limit the numbers of electrodes within the constrained fascicle ( ), especially when considering inflammatory response characteristics ( ), which will further reduce the intimacy of contacts with axons. Prior short-term applications of intrafascicular approaches showed increasing stimulation thresholds ( ). The number of reported sensory locations and perceptions elicited was similar to long-term extraneural approaches ( ). The functional performance of an intrafascicular approach is similar to the extraneural approach ( ).
Stimulation Parameters and Patterns
In addition to physical proximity, a second powerful tool that is often not considered is the manipulation and design of the spatiotemporal electromagnetic field. Multiple electrical contacts in electrodes like the FINE ( ) allow for the summation of electrical fields to shape and move areas of activation within the nerve. Therefore, the sensory locations are not limited to the number of electrical contacts. It has been demonstrated clinically that additional sensory perceptions are possible ( ) and the number of additional sensations possible is an active area of research.
Pulse shapes . The magnitude of the electrical fields decay by a factor of 1/ r , where r is the distance from the electrode. Nerve activation is proportional to the rate of change of the voltage difference along the axon ( ). Hence, with simple square pulses, closer, larger, myelinated axons will be activated before smaller, distant, and unmyelinated axons. However, axonal excitation is also controlled by the nonlinear dynamics of the membrane channels ( ) that can be manipulated by changing the shape of the pulse. Types of waveforms that have been explored include quasitrapezoidal pulses designed to recruit small axons before the large ( ) and ramp pulses to activate more distal axons before closer axons ( ). To minimize the power requirements for implanted devices, exponential-shaped waveforms activate the larger and closer axons in the same order, but with minimized energy ( ).
Frequency modulation . Axons convey information by spikes across a population of axons. In any single axon, the information is carried by the spike rate or frequency. Bursting pulse trains activating a small population of neurons can change the quality of tactile perceptions ( ). Changing stimulation frequency in somatosensory applications is related to intensity ( ). In studies with intact hands, increasing the intensity of either mechanical pressure or vibration results in (1) an increase in the firing rate of nerve fibers with receptive fields in the center of the mechanic stimuli and (2) the recruitment of fibers with nearby receptive fields ( ). With electrical stimulation, it is possible to separately vary stimulation frequency and number of fibers recruited. Using this model, it is shown that intensity is encoded in the total spike rate ( ). The total spike rate is related to the magnitude of the stimulation strength above threshold multiplied by the frequency of the stimulation. This is call the activation charge rate (ACR, Eq. 103.1 ).
ACR=fstim(Qstim−Qthr)
ACR = f stim ( Q stim − Q thr )
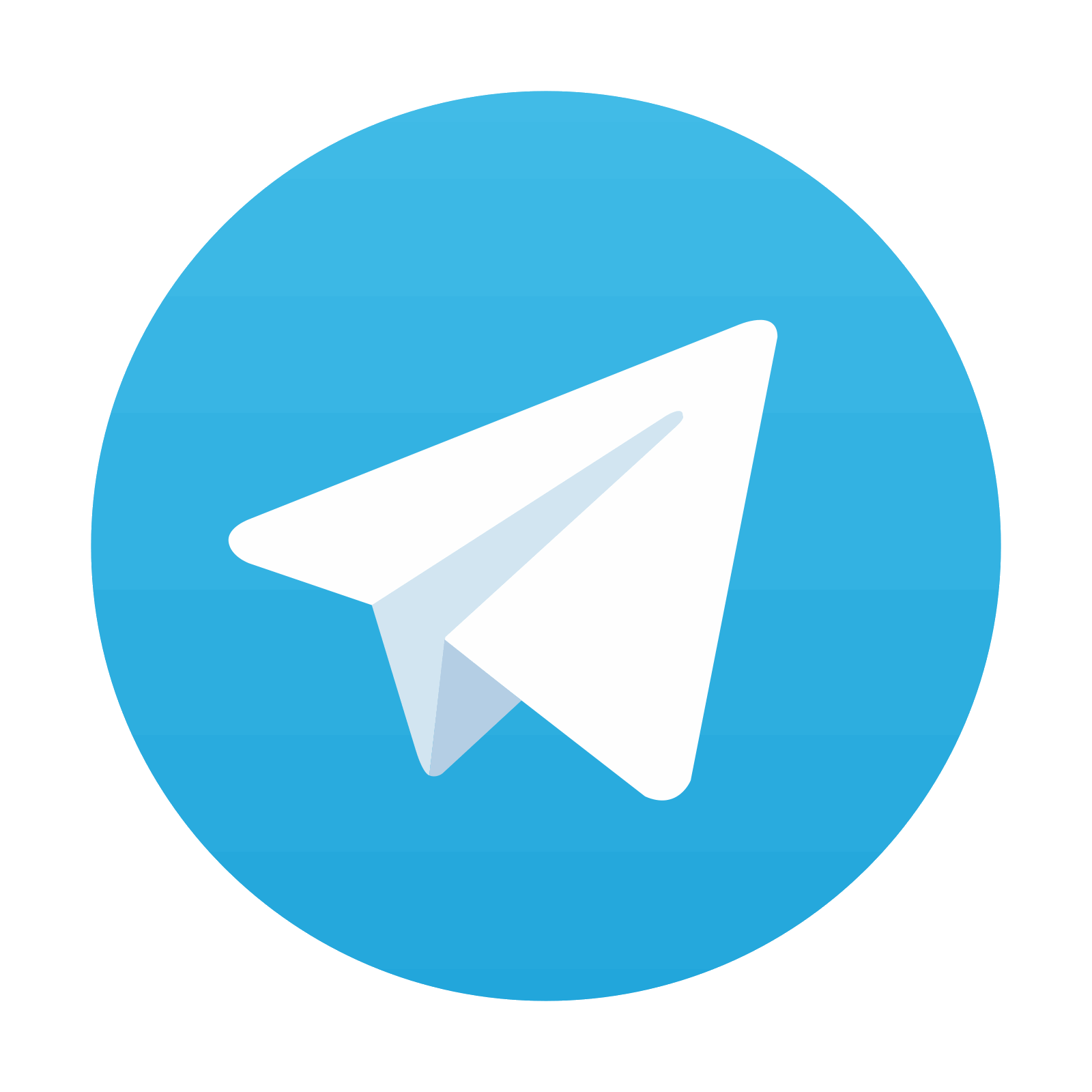
Stay updated, free articles. Join our Telegram channel
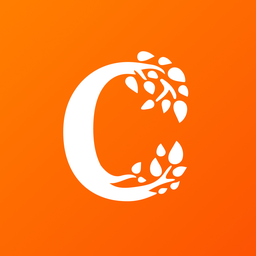
Full access? Get Clinical Tree
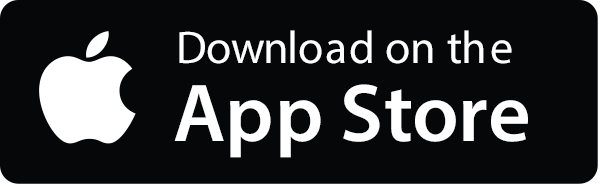
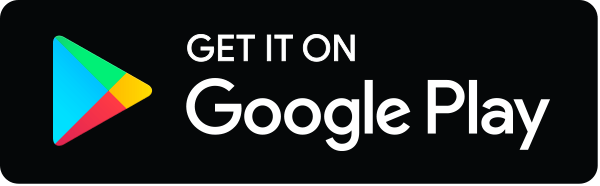