CHAPTER 94 Neuroprosthetics
Neural Interface Systems
The notion of establishing a connection between brain and machine for therapeutic benefit is not entirely new. By the early 1950s, stimulating and recording electrodes were placed intracranially in human cortex in the hope of alleviating psychosis.1 As technology advanced, it adapted to the growing understanding of neurological disorders. Recent multidisciplinary research has pursued the development of a fully integrated NIS to restore function and markedly improve quality of life. An NIS is an innovative means of coupling physical devices capable of interacting with the central nervous system (CNS) with devices that interact with sensory stimuli or motor prostheses. As a result, NISs have enormous potential to benefit the substantial patient population suffering from neurological disorders that leave the central structures intact, such as motor neuron disease, spinal cord injury, stroke, and most causes of congenital and acquired deafness and blindness. In fact, some neurotechnologies have already experienced clinical success. Cochlear implants have become a routine therapeutic measure for restoring audition to the hearing impaired, and deep brain stimulation (DBS) is increasingly being used as a treatment option for symptoms of Parkinson’s disease, dystonia, and even depression.2–5 These stimulating technologies are examples of devices generally included in the growing field of neuromodulation.
An NIS can may communicate information into or out of the nervous system. A neuromodulatory sensory prosthesis, or an input NIS, is a read-in system in which information is transduced from a physical device, such as a microphone or camera, to neural tissue. In contrast, an output NIS is designed to read out neural signals from the brain to provide movement signals or a readout of brain function. DBS is not included here because it is thoroughly covered in other chapters and is a different type of NIS (not a true sensory NIS). The standard components of both input and output NISs include a signal sensor to record neural or environmental signals (e.g., spikes, sound, or light), a signal-processing algorithm to decode information from the CNS or encode information from the environment, and a device (such as a stimulating electrode or assistive technology) to transform output signals into action (Fig. 94-1). Thus, one can think of these systems in the context of two broader categories: those that interpret signals and those that create them. An output NIS provides a new route for disconnected cortical motor signals that are unable to reach muscles so that computers, prosthetic limbs, and even the user’s own muscles can be controlled with functional electrical stimulation (FES). For an input NIS, such as cochlear implants and visual prostheses, information from the surrounding environment is encoded into patterned electrical stimulation of intact neural tissue.
Neuromotor Prostheses
Several hundred thousand people currently suffer from paralyzing disorders in which the brain areas responsible for volitional movement are preserved but the brain is disconnected from output pathways or the muscles necessary to enact movement.6 Paralysis of this sort can result from various sources at different levels of the motor system. Upper level motor areas are frequently preserved in cases of trauma, stroke, and neurodegenerative diseases of the descending motor tracts and spinal cord.7 Central motor control areas are also preserved when the absence of functional muscles results in immobilization, such as with amputation or muscular dystrophy. An NIS can replace or restore these lost motor functions by extracting movement-related neural signals from the cerebrum, decoding them, and using the information to accurately control an output device, such as a prosthetic limb.
Useful Neural Signals
To ensure precise control of NISs, movement-related neural signals must be information rich, have a high information transfer rate, be reliable, and be safely accessible by modern recording devices. One natural source for acquiring movement information is the frontal lobe motor areas where these commands are generated. The primary motor cortex (M1), which is located roughly along the posterior half of the precentral gyrus, and the premotor cortex and supplemental motor area, which extend anteriorly from M1, give rise to the neural signals responsible for planning, initiating, and generating volitional movement.8–10 Neuromotor prosthesis research has largely concentrated on restoring function to the upper extremities, where the somatotopic relationships for arm and hand motion have been particularly well defined by myriad neurophysiologic methods.11 There remains some disagreement in the field on which signal types originating from these regions are best for controlling neuromotor prosthetics, and this is an area of active exploration in basic research in nonhuman primates.
There are essentially two types of electrical signals in the brain: action potentials (spikes) and field potentials (FPs) (Fig. 94-2). Both categories, detected by different sensor technologies, are currently used as control signals for neuroprosthetic devices. NISs that use FPs are attractive because FPs can be acquired noninvasively with scalp electrodes whereas systems interpreting spiking activity require electrodes implanted in the brain parenchyma. As a result of the inherently invasive nature of decoding spike information, NISs using this approach have only recently been used in humans. Some NIS researchers are also developing systems that record FPs with epidural or subdural electrodes to obtain more reliable signals.
The action potential is the fundamental information-carrying unit of the nervous system.12 NISs can obtain rich movement-related information from the spike rate–based code of individual neurons. The basis for accurate control in NISs is that the firing patterns of neurons in the motor cortex reflect the direction of intended reach.13,14 In addition, firing activity in cortical motor neurons has been shown to reflect hand position, velocity, forces, joint angles, and target goals.15 Solely by recording and decoding these signals, the path of hand motion can be determined with decoding software and represented by an on-screen cursor or a prosthetic limb. Numerous experiments in nonhuman primates first illustrated that subjects could accurately modulate M1 neural output to drive computer cursor motion in two- and three-dimensional visuomotor tasks.16,17 These experiments served as the basis for trials in human subjects. The first implanted electrode for the purpose of driving an NIS consisted of two wires in a conical glass electrode.18 Even with this rudimentary implant, the patient was able to control a binary switch with the NIS output. In 2004, a team of Brown University researchers launched the BrainGate pilot trial, which demonstrated the feasibility of using an NIS in tetraplegic human participants. Important for the prospects of NIS development, this group found that directional tuning of neurons in M1 is present years after a patient has become paralyzed from spinal cord injury or brainstem stroke.19,20 Control of these signals was reportedly natural (cursor movement corresponding to intended hand or arm movement), required only a short period of training, and provided users with several degrees of freedom, including discrete functions similar to clicking a mouse button.21,22
The other type of neural electrical signal capable of controlling an NIS is FPs, many of which can be recorded noninvasively. FPs are much more complex signals than action potentials in that they represent the summed transmembrane currents across groups of neurons with different properties. Although this signal arises from a wide distribution of neurons, FPs have long been understood to carry significant information in their oscillations. Although spikes represent a discrete, binary neural signal, FPs carry both a neural activity–related signal and a brain state signal, which is dependent on higher cognitive functioning, alertness, and sleep stage.23 FPs can be classified according to their two characteristic signal band types: event-related potentials and rhythmic signals (see Fig. 94-2).20 Event-related potentials are the sweeping potential changes across a large population of neurons that are evoked by external events or volitionally. The P300 wave, which entails a signature voltage deflection approximately 300 msec after the stimulus event, is a prominent signal used for NISs. This signal is regarded as a sign of higher cognitive processing because it represents the rapid integration of sensory stimuli within the cortex. Rhythmic FPs can be further subdivided into fast, medium, and slow frequencies, all of which can serve as control signals for NISs. Humans can use these rhythms to control NISs by learning how to modulate the frequency or amplitude of these potentials. In the first successful human electroencephalography (EEG)-based NIS, participants modulated slow rhythms (less than 1 Hz), such as the Bereitschaftspotential, to achieve continuous, two-dimensional control.24,25 Despite this success, interest is shifting toward faster FP rhythms because they contain more information and are more easily modulated by the user. Some experiments have begun to evaluate medium rhythms, including alpha (8 to 12 Hz) and beta (12 and 20 Hz or greater) frequency bands. A more natural NIS control signal is generated because midrange rhythm amplitudes can be changed with the imagination of movements.25 FPs with the highest frequencies, fast beta rhythms and gamma rhythms (30 to 100 Hz), appear to contain distinct, specific information. For example, the beta rhythms in M1 in paralyzed humans and nondisabled monkeys have been found to indicate the transition from movement planning to execution.19,26 Gamma oscillations may provide additional dimensions to an FP NIS control signal inasmuch as they have been correlated with spiking activity in the parietal and visual regions of the cortex.27 Local FPs represent the highest frequency FPs and are thought to contain information about populations of spiking neurons.
Signal Sensors and Their Limitations
A range of sensors are available for capturing neuronal activity to use as an NIS control signal; each has certain advantages and limitations (Fig. 94-3). Noninvasive strategies use scalp electrodes and thereby avoid the risks associated with surgery. These external sensors record an EEG signal consisting of lower frequency oscillations. Although this EEG setup is relatively simple, the signals are not only attenuated by the skull and scalp but are also limited in their bandwidth. Higher frequency FPs and neural spiking activity are not accessible, which limits the NIS control signal. In contrast, by invasively recording epidurally or subdurally, an electrocorticography (ECoG) signal can be acquired, which has higher fidelity than EEG signals and is less prone to artifact. There is the potential for using ECoG in conjunction with subdural grid mapping in pre-resection epilepsy cases, where signals can be used from multiple electrodes. ECoG for NIS applications will require an invasive procedure, but it is hoped that the associated risks can be decreased as techniques improve and devices shrink. No ECoG-based NIS has yet been tested in humans with paralysis to determine whether ECoG signals from a limited area of cortex can serve as a reliable control signal for neural prosthesis applications.
Invasive strategies are currently the only way to record spiking activity from individual neurons. However, one of the concerns with penetrating electrodes is the neuroimmune response elicited. Encapsulation is a common feature of the response as glia react to the foreign electrode. The degree of encapsulation is highly variable and may depend on the state of the patient’s immune system, the fabrication techniques of the penetrating electrodes, the insertion technique, and the materials with which the electrodes are constructed. Recording and stimulation with these electrodes are not necessarily precluded by gliosis; in fact, some electrode designs take advantage of the response from local cell populations. These electrodes are coated with neurotrophic factors that promote migration of neurites toward the electrode and thereby produce a longer-lasting and tighter coupling between stimulation and neuronal response.18 Another drawback of invasive recording techniques is the requirement for a percutaneous connection to external components (Fig. 94-4), which increases the risk for infection. Although biocompatibility is a major concern, preliminary studies in humans have shown that implanted electrodes are in fact safe and can provide signals for many years.21
Decoding Movement Signals
Many algorithms have been proposed for decoding spike-based data. Most firing rate–based decoding methods rely on data from a large population of neurons, or “units.” Population-based decoding more closely replicates the way we believe the nervous system naturally encodes movement information. Furthermore, ensemble-based decoding is preferred because it provides a more stable output signal; the population code is less susceptible to noise and extreme deviations in activity.28 Decoding then works by analyzing the specific multiunit pattern of activity across the neuronal population and comparing it with a known reference pattern distribution, which is usually generated at the beginning of a recording session. When the decoding algorithm is being built, the user is told to imagine moving a hand in a given manner, and the software learns to correlate that pattern of neural activity with the given motion. Decoding algorithms can use a linear filter or a recursive, bayesian inference decoding algorithm. In a linear filter system, individual units are assigned different weights, which correlate spiking with the direction and velocity of movement. In bayesian inference filters, such as the Kalman filter, the decoder is continuously updating by recursively incorporating position, velocity, and acceleration information about trained units to predict and control the movement kinematics of the output signal. In either case, the neural firing data on which the algorithm is trained must resemble the neural activity during user control tasks for stable neural cursor control to occur.
In an EEG-based NIS, decoding of FP signals relies on the user’s ability to learn to modulate slow cortical potentials or to selectively control a particular frequency band of the EEG at a particular electrode location (or locations).29 Decoding algorithms can be simple (linear) or complex (neural networks), but in either case a feature such as amplitude must be extracted from the signal synchronicity and correlated with a particular output action or direction.30 Other devices exploit the involuntary evoked potentials that appear in the presence of external stimuli (i.e., the P300). This state signal is extremely useful and can be decoded as a click signal.
Output Devices
Once a reliable movement output signal has been established, a wide range of prosthetic effector devices can be controlled. The goal of neuromotor prosthesis development is to create a system that restores, assists, or replaces the capabilities of natural movement to individuals disabled by a variety of nervous system disorders. Because the output signal from NISs is very versatile, it is possible that these implants could supply control signals for devices that provide unnatural abilities, such as control of remote robots.31
Assistive Technologies
Computer Cursor
Continuous point-and-click control of a computer cursor is possible in tetraplegic individuals by using intracortical NISs, and it offers nearly infinite possibilities for improving patient independence and quality of life. In the BrainGate NIS trial, multiunit spiking activity in M1 was modulated by the participants to control a cursor for opening e-mail, browsing the Internet, operating a television, using a drawing program, and playing video games.19 Importantly, this control was natural and required no learning. The participants simply imagined moving their arm or hand in the desired direction and the cursor moved with remarkable accuracy. Recently, one participant studied over the course of three sessions successfully reached and clicked 96% to 100% of specified targets.22 Some noninvasive methods have also been devised for point-and-click cursor control, but control is less intuitive (using different EEG signals) and reliable than with an intracortical NIS in humans. Furthermore, control of closed-loop spike-based NISs (i.e., visual feedback of cursor movement) can more easily be stabilized because the user can more intuitively modulate the intended movements to obtain accurate cursor control. As signal decoding improves, a tetraplegic patient will one day be able to operate all the computer software available to able-bodied individuals solely by using a neural control signal.
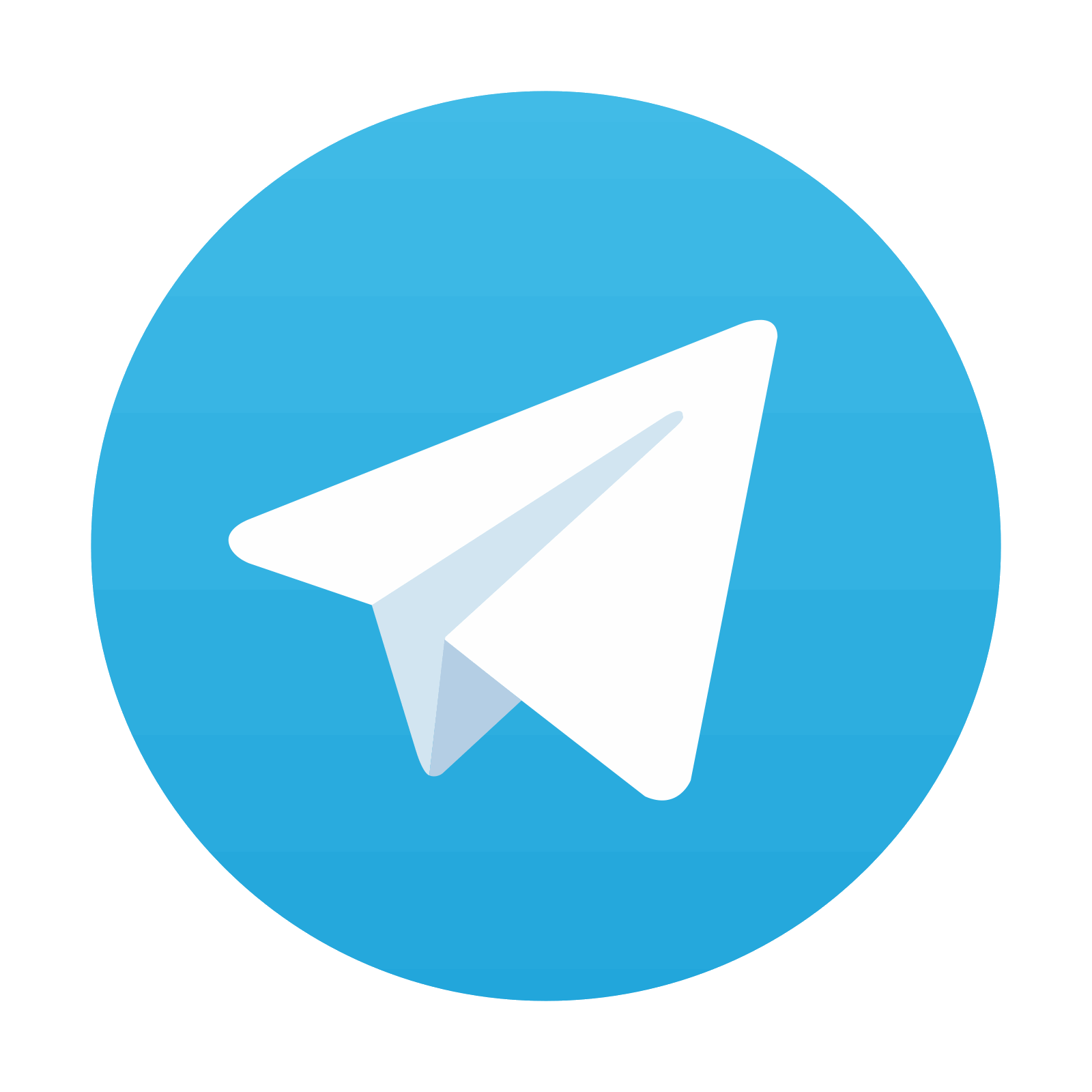
Stay updated, free articles. Join our Telegram channel
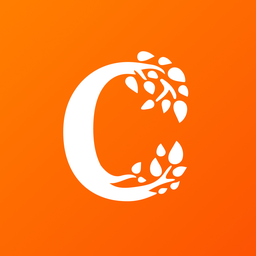
Full access? Get Clinical Tree
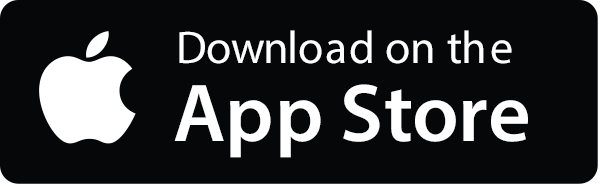
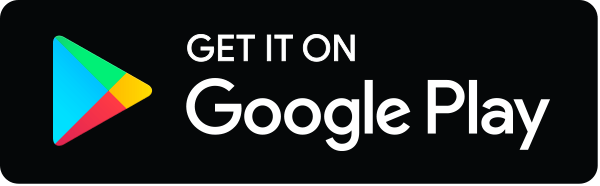