Normal EEG and Sleep: Adults and Elderly
Bernard S. Chang
Donald L. Schomer
Ernst Niedermeyer
INTRODUCTION
There is perhaps nothing as compellingly beautiful to a clinical neurophysiologist as the appearance of a normal adult elec-troencephalogram (EEG) recording, in wakefulness and sleep. But the relative order, symmetry, and transitions that populate such a recording and are so welcome to the clinically trained eye can lead us to forget the fundamentally remarkable phenomena that electrical activity from the human brain can indeed be measured routinely by electrodes placed only on the scalp; that defined, well-catalogued abnormalities in the resulting measurements can be identified and interpreted with clinical meaning; and that our entire understanding of this field has essentially arisen within only the past century.
A chapter on the normal EEG is actually more difficult to organize than it might seem. Many clinical elec-troencephalographers would define normality on an EEG as being simply the absence of identifiable abnormalities. In other words, there is no single rhythm, feature, or characteristic of an EEG that must be present to make it normal (except, perhaps, reactivity), and, indeed, no combination of any such findings either. Such is the nature of human EEG, and this must be kept in mind in the clinical interpretation of tracings. Having said that, decades of EEG study, beginning with Berger’s early fundamental descriptions and continuing now with advanced power spectral analyses, have shown us that there are certain basic recognizable patterns, seen at least some of the time in almost all healthy individuals, that together constitute what we would consider to be representative of a normal EEG. It is the goal of this chapter to define and describe these recognizable elements, with attention to their clinical implications and their significance for our understanding of underlying cerebral physiology.
EEG FREQUENCIES
The EEG contains a wide frequency spectrum, but it is not simply a hodgepodge of frequencies. Rhythmicity seems to create some law and order among waves of various lengths and amplitudes. The impression of prevailing rhythmicity and organization, however, is not a yardstick for the normality of an EEG. Pronounced rhythmicity may be a sign of abnormality, and a chaotic appearance does not necessarily imply abnormality. Reactivity may be the magic word in such cases; an EEG of mixed frequencies may be quite responsive to certain stimuli.
The frequency range of the EEG has a fuzzy lower and upper limit. There are ultraslow and ultrafast frequency components that play no significant role in the clinical EEG, with the exception of ultraslow activity in profound coma and near-terminal states. For these reasons, the frequency-response curve of an EEG apparatus concentrates on the clinically relevant frequency range, which is also the most important from the psychophysiologic viewpoint. This range lies between 0.1/sec (or cycles per second [cps] or Hz) and 100/sec and, in a more restricted sense, between 0.3 and 70/sec. In the normal adult, the slow ranges (0.3 to 7/sec) and the very fast range (above 30/sec) are sparsely represented; medium (8 to 13/sec) and fast (14 to 30/sec) ranges predominate.
These frequencies are broken down into the following bands or ranges:
Delta below 3.5/sec
Theta 4 to 7.5/sec
Alpha 8 to 13/sec
Beta 14 to 30/sec
Gamma above 30/sec
The sequence of these Greek letters is not logical and can be understood only in the historical view. The terms alpha and beta rhythm or waves were introduced by Berger (1); the term gamma rhythm was subsequently used by Jasper and Andrews (2) to designate frequencies above 30 or 35/sec; these were essentially 35 to 45/sec and superimposed on the occipital alpha rhythm (see Ref. 3). This term was temporarily abandoned, and gamma frequencies became a part of the beta range.
The use of the term gamma rhythm or gamma frequency range made an impressive comeback during the 1990s. The use of a fast beta range and a very fast gamma range might have been convenient for those who utilize frequency analysis with power spectra. Furthermore, EEG rhythm research in the 1990s unearthed the all-but-forgotten term gamma rhythm (4, 5, 6 and 7). In recent EEG rhythm research, rhythmic activities of the brain are often conceived mainly as induced rather than as spontaneous rhythms.
The term delta rhythm was introduced by Walter (8) to designate all frequencies below the alpha range. Walter himself, however, found a need to introduce a special designation for the 4 to 7.5/sec range and used the letter theta. He thus bypassed the Greek letters epsilon, zeta, and eta; he chose theta to stand for thalamus because he presumed a thalamic origin of these waves (also see Ref. 9).
The term pi rhythm has been used for the designation of posterior slow rhythms (3 to 4/sec) without harmonious relationship to the posterior alpha rhythm according to Dutertre (3) who recommended the preferable (although certainly less precise) term posterior slow rhythms. Hardly anyone has used the term pi rhythm since the 1990s.
The term phi rhythm was suggested (according to Ref. 10) for the designation of monorhythmic posterior delta waves (less than 4/sec), distinct from the background and occurring within 2 seconds of eye closure. This rhythm was also described by Belsh et al. (11) as “posterior rhythmic slow activity after eye closure.”
The alpha-like anterior temporal kappa rhythm (12) is a controversial pattern that is discussed later in this chapter. Kugler (13) has been using the term sigma activity instead of sleep spindles and, furthermore, the term sigma rhythm for activity in the 11 to 15/sec range. The term rho waves was used for the activity known as positive occipital sharp transients of sleep (POSTS) (14), but it has disappeared.
Other Greek letters have been proposed for the designation of distinct EEG activities. Mu rhythm and lambda waves are discussed in this chapter. The term tau rhythm denotes a physiologic alpha rhythm of the temporal region (perhaps identical with the “third rhythm” discussed in this chapter). “Zeta wave” simply denotes a certain type of delta wave (rather than a rhythm) with some sharp configuration (15,16).
Thus, 14 of the 23 letters of the Greek alphabet are being used in the EEG terminology, and this number could be even higher. In our opinion, it might be better to limit the Greek terms to the classical EEG frequency ranges retaining solely the letters alpha, beta, gamma, delta, and theta.
EEG AMPLITUDES
The EEG denotes voltage plotted against time. The voltage of the EEG signal determines its amplitude. The passage of the cortical EEG signal through leptomeninges, cerebrospinal fluid, dura mater, bone, galea, and scalp has a strongly attenuating effect on the original signal (17). Corticographic discharges show amplitudes of 500 to 1500 µV (0.5 to 1.5 mV) and several millivolts in prominent spiking. The amplitudes of the scalp EEG are markedly reduced and lie between 10 and 100 µV (in adults, more commonly between 10 and 50 µV).
The EEG amplitudes are measured from peak to peak. Precise determination of the voltage of each wave is unnecessary and should be discouraged as pseudoaccuracy; too many variables are involved (above all, the interelectrode distance and the type of montage, whether bipolar or referential recording). Electroencephalographers may indicate in their reports a certain amplitude range, such as “alpha rhythm from 20 to 30 µV,” or, even better, limit themselves to statements such as “of medium voltage” or “of low to medium voltage.”
A given frequency can be rendered abnormal by excessive voltage. This is true for all frequencies, and it is particularly important for the fast (beta) band. The problem of low voltage will be thoroughly discussed, because low amplitudes can indicate a life-threatening decline of cerebral voltage output, whereas the vast majority of low-voltage records are “desynchronized” (discussed later) and are a variant of normalcy.
BASIC EEG PATTERNS SEEN IN WAKEFULNESS
Alpha Rhythm
Definition
The International Federation of Societies for Electroencephalography and Clinical Neurophysiology (IFSECN) (committee chaired by G. E. Chatrian; see Ref. 18) proposed the following definition of alpha rhythm (Fig. 11.1):
Rhythm at 8 to 13 Hz occurring during wakefulness over the posterior regions of the head, generally with higher voltage over the occipital areas. Amplitude is variable but is mostly below 50 µV in adults. Best seen with eyes closed and under conditions of physical relaxation and relative mental inactivity. Blocked or attenuated by attention, especially visual, and mental effort (18).
This committee also has pointed out that the term alpha rhythm must be restricted to rhythms fulfilling all of the above criteria. Rolandic mu rhythm may have the same frequency range, but its topography and reactivity are different (also see Ref. 19).
Frequency
EEG maturation shows the gradual frequency increase of a posterior basic rhythm that is detectable around the age of 4 months with a frequency of approximately 4/sec. This posterior dominant rhythm shows a progressive frequency increase with
average values of around 6/sec at the age of 12 months and 8/sec at the age of 3 years. At that time, the alpha frequency band is reached, and there is justification for the use of the term alpha rhythm. The frequency reaches a mean of about 10/sec at the age of 10 years. This is essentially the mean alpha frequency of adulthood; in other words, the progressive alpha rhythm acceleration usually ends around the age of 10 years, but the second decade of life (and to some degree also the third decade) features a constant decline of intermixed posterior slow activity that is usually present in considerable quantity at the age of 10 years.
average values of around 6/sec at the age of 12 months and 8/sec at the age of 3 years. At that time, the alpha frequency band is reached, and there is justification for the use of the term alpha rhythm. The frequency reaches a mean of about 10/sec at the age of 10 years. This is essentially the mean alpha frequency of adulthood; in other words, the progressive alpha rhythm acceleration usually ends around the age of 10 years, but the second decade of life (and to some degree also the third decade) features a constant decline of intermixed posterior slow activity that is usually present in considerable quantity at the age of 10 years.
The frequency of the alpha rhythm tends to decline in elderly individuals. This decline apparently reflects some degree of cerebral pathology, which may be vascular or degenerative, in many instances. Healthy and vigorous elderly people may show little or no alpha frequency decline, even in the ninth decade.
The figure of 10.2 ± 0.9/sec has been indicated as the mean adult alpha frequency (20). An element of instability of the alpha frequency must be taken into consideration; according to Townsend et al. (21), the alpha rhythm frequency can be stabilized by sinusoidally modulated light. Extreme upward gaze tends to facilitate the posterior alpha rhythm (22,23). Lateral eye deviations may have similar effects (24).
An alpha rhythm frequency shift to the faster portion of the band is not uncommon and is essentially within normal limits, as will be discussed later. The similarities between the frequencies of alpha rhythm and the physiologic finger tremor have been discussed by Isokawa and Komisaruk (25). Immediately after eye closure, the alpha frequency may be accelerated for a moment (“squeak effect,” after Ref. 26).
Amplitude
Alpha rhythm amplitudes vary considerably from individual to individual and, in a given person, from moment to moment. The electroencephalographer, therefore, should look for stretches of optimal alpha output. A referential montage to the ipsilateral ear is usually most suitable for the determination of the alpha rhythm amplitude, but the interelectrode distances must always be considered. The maximum alpha voltage is usually over the occipital region as such, but a bipolar montage with a parasagittal array may obscure rather than reveal the true alpha maximum. The alpha amplitude may be quite small in the channels displaying P3-O1 and P4-O2 because of massive homophasic activity, which results in canceling out.
The alpha amplitudes tend to show constant waxing and waning. For this reason, trains of alpha waves show a typical spindle shape with a belly and a thin portion. However, the term spindle has been reserved for a classical pattern of sleep and should not be used in this context.
Berger (1) found alpha rhythm voltages of 15 to 20 µV; these are small values when one considers his fronto-occipital recording technique; the smallness was probably due to the limitations of his Edelmann string galvanometer. According to Cobb (27), the alpha rhythm voltage fluctuates between 0 and 40 to 50 µV in the individual record; values above 100 µV are uncommon in the adult, whereas maximums of 5 to 10 µV are frequently seen (27). The work of Simon (also known as Simonova) and her coworkers has shed more light on this subject; Simonova et al. (28) found amplitudes between 20 and 60 µV in 66% of their subjects; values below 20 µV were found in 28% and above 60 µV in 6% (also see Ref. 29).
Higher alpha amplitudes are more likely to be found in association with slower alpha frequencies (30,31). There is good evidence of a mild-to-moderate alpha amplitude asymmetry with a higher voltage on the right (20,27,29,31, 32 and 33). This seems to indicate that the alpha rhythm is of greater amplitude over the nondominant hemisphere, but no convincing conclusion concerning handedness can be derived from this asymmetry (20). This physiologic asymmetry has been confirmed by Matousek et al. (34), who also found a reversal of this rule (i.e., higher voltage on the left occiput in patients with endogenous depression).
Amplitude asymmetries must be demonstrated in both referential and bipolar montages from two or more posteriorly placed electrodes (such as parietal and posterior temporal) before they are considered significant (19). It may be difficult to distinguish between physiologic and truly abnormal alpha amplitude asymmetries.
Morphology
The alpha rhythm is usually characterized by rounded or sinusoidal wave forms. However, a sizable minority of individuals have sharp alpha configuration. In such cases, the negative component appears to be sharp and the positive component appears to be rounded, similar to the wave morphology of rolandic mu rhythm.
The sharp configuration of posterior alpha waves is by no means an abnormality. It is a common finding, especially in young adults, adolescents, and older children. An admixture of beta waves is usually the cause of the sharp configuration; drug effects from sedatives or minor tranquilizers must sometimes be suspected in such cases.
Spatial Distribution
The alpha rhythm is clearly a manifestation of the posterior half of the head and is usually found over occipital, parietal, and posterior temporal regions. This observation of Adrian and Matthews (35) was doubted by Berger (36), whose concept of alpha rhythm as a global cerebral rhythm was an erroneous conclusion from his fronto-occipital bipolar recording technique. The alpha rhythm may extend into central areas, the vertex, and also the midtemporal region. When the central region is strongly involved, the alpha rhythm must be distinguished from possibly coexisting rolandic mu rhythm. This is usually easily demonstrable with eye opening, which should lead to attenuation of the alpha rhythm but not the mu rhythm.
The alpha rhythm may occasionally extend slightly into the superior frontal leads (F3, F4). Extension into the frontopolar region (Fp1, Fp2) is practically unheard of. Apparent alpha rhythm in the frontopolar leads may be very prominent in referential (unipolar) montages if the referential ear electrode picks up the posterior alpha rhythm. This is particularly common when the mastoid region is used instead of the ear lobe (the mastoid being a preferred place with paste technique).
Another source of confusion is eyelid flutter, with closed eyes giving rise to frontal artifacts in alpha frequency.
Another source of confusion is eyelid flutter, with closed eyes giving rise to frontal artifacts in alpha frequency.
In depth electroencephalography and with occipital implants, posterior alpha rhythm can be demonstrated throughout the depth of the occipital lobe and even in the vicinity of the lateral geniculate body. According to Albe-Fessard (37), alpha rhythm may be recorded from the medial pulvinar but not from the remaining thalamic nuclei in the human (also see Ref. 38). In the dog, Lopes da Silva et al. (39) demonstrated that alpha rhythm of the same peak frequency, bandwidth, and reactivity can be recorded from the visual cortex as well as from the visual thalamus (lateral geniculate body, pulvinar nuclei).
Reactivity
The posterior alpha rhythm is temporarily blocked by an influx of light (eye opening), other afferent stimuli, and mental activities. The degree of reactivity varies; the alpha rhythm may be completely blocked, suppressed, or attenuated with voltage reduction. The alpha blocking response to eye opening was discovered by Berger and described in his first report (1) on the human EEG; it came as a great surprise for investigators who were searching for action potentials and hence would have expected enhancement of EEG voltage with influx of light. Berger’s own explanation was the concept of a zone of inhibition surrounding the area of excitation by the afferent stimulus (40,41).
It is noteworthy that in some subjects the alpha suppression is more pronounced than in others. These persons may have an alpha-free stretch of desynchronized and chiefly fast EEG activity for the duration of the eye opening, even in rather dim light. In others, the alpha blockage lasts for less than 1 second. According to Gibbs and Gibbs (42) “after the eyes have been held open for a few minutes, low voltage alpha waves … usually reappear, unless the subject continues to look at something which holds his interest.” The amplitude ratio between eyes closed (well-developed alpha) and eyes open (beta of much smaller voltage) declines with advancing age (43).
Alpha attenuation due to auditory, tactile, and other somatosensory stimuli or heightened mental activity (such as solving difficult arithmetical problems) is usually less pronounced than the blocking effect with eye opening. Indeed, the blocking or attenuating effect of mental arithmetic on the posterior alpha rhythm may not be present in most cases (44). In a study done in 1280 patients (598 with normal EEG tracings, 682 with various degrees of EEG abnormality), alpha suppression or attenuation with calculation (serial sevens) was noted in only 21 (1.6%) of the patients. On the other hand, Berger (40,45, 46, 47 and 48) showed fine examples of arithmeticinduced alpha blocking. A possible explanation for the discrepancy lies in the difficulty of the task: the arithmetical problems given to Berger’s two teenage children, Klaus and Ilse, were more challenging. Other factors may also be at work: even simple arithmetic may cause alpha blocking if the subject shows great motivation trying to please the examiner. With a nonchalant approach, there is usually no alpha blocking.
No EEG is complete without certain reactivity tests. Although alpha rhythm disappears with the earliest approach of drowsiness, there are exceptional cases of persisting posterior alpha rhythm in profoundly comatose patients with pontine vascular lesions; their alpha rhythm shows no reactivity, even with strong nociceptive stimuli. According to Kiloh et al. (33), “complete unresponsiveness of the alpha rhythm to visual stimuli is a rare and unequivocally abnormal finding.”
Interindividual Differences
When Adrian and Matthews demonstrated their own EEGs on May 12, 1934, at a meeting of the astonished members of the Physiological Society in Cambridge, England, it was found that Adrian’s 10/sec alpha rhythm was quite impressive, whereas Matthews produced “no regular waves” (49). Studies of further subjects showed that Adrian’s alpha development was that of the majority, whereas Matthews, the ingenious engineer and designer of Adrian’s instrumentation, belonged to a minority of persons with little or no organized alpha rhythm.
Davis and Davis (50) distinguished four types of records: (a) dominant alpha (found in 20% of healthy adults); (b) subdominant alpha (35%); (c) mixed alpha (20%); and (d) rare alpha (25%). Golla et al. (51) distinguished three alpha types: M for minus or minimal, P for persistent, and R for responsive. The P type shows no real persistence of alpha, but has a very short blocking response to eye opening (also see Ref. 33). Another type of alpha rhythm is the “monotonous high voltage alpha,” which shows little or no waxing and waning of the amplitude (52). These alpha traits may be, to some degree, genetically transmitted (50,53,54); marked similarities have been reported in identical twins. Small differences in the EEG of males and females have been reported by Veldhuizen et al. (55).
A detailed review of historical EEG literature on the alpha rhythm inevitably uncovers multiple publications suggesting a relationship, or lack thereof, between alpha rhythm traits and particular personality features (56, 57, 58, 59, 60 and 61), with little evidence of reproducible findings. Similarly, attempts at simple correlations between alpha rhythm and intelligence have largely been futile or unconvincing (36,45,62, 63, 64, 65, 66 and 67), but with the use of power spectral analysis, Gasser et al. (68) found good correlations between alpha rhythm (plus other EEG criteria) and intelligence, and individuals who are faster in retrieving information from memory tend to have higher alpha rhythm frequencies (69).
Intraindividual Differences
A person’s EEG traits and alpha rhythm development must not be considered as permanent and unchangeable features like fingerprints. Every EEG tracing of some length gives testimony to a certain degree of variability, even within the state of relaxed vigilance. This is probably the effect of a large number of physiologic and psychophysiologic variables. To mention just one example, the waxing phase of alpha amplitudes has been related to the waning phase of afterimages (70). With advancing age, the alpha frequency tends to decrease (71) but this is probably the effect of cerebral pathology (vascular or degenerative) occurring at old age.
Circadian studies of the EEG have been done sparingly (72,73) and with inconclusive results (for further data, see Ref. 74). The menstrual cycle has been thought to influence the EEG and especially the alpha rhythm frequency; Harding and Thompson (74) have summarized the work in this field as follows:
Preovulatory phase (days 5 to 14): Alpha frequency increased, amount of beta increased, photic driving reduced.
Postovulation or luteal phase (days 15 to 23): Alpha slower, amount of alpha increased, less beta and more theta activity, photic driving increased.
Premenstrual phase (days 23 to 28): Alpha frequency increased, amount of alpha reduced, more beta, less theta activity.
Menstrual phase (days 1 to 5): Alpha frequency slowed and amount increased, less beta, more theta activity.
Relationship to Vigilance and Anxiety
Alpha rhythm is the classical EEG correlate for a state of relaxed wakefulness best obtained with the eyes closed. A degree of higher alertness attenuates or suppresses the alpha rhythm, which is then supplanted by “desynchronized” low-voltage fast activity.
The earliest stage of drowsiness is characterized by “alpha dropout” (Fig. 11.2). The trains of alpha waves become less and less continuous, and the last alpha fragments finally give way to a low-voltage pattern of mixed slow (mostly theta range) and fast frequencies. This type of alpha dropout is a hallmark of a
normal adult EEG; in children and infants, various types of slow patterns appear. In adults with neurodegenerative disease, a posterior alpha rhythm of normal appearance may be replaced by activity in the theta and delta range; a special pattern with rhythmical widespread (but mainly parietotemporal) 5 to 7/sec activity has been individualized recently by Westmoreland and Klass (77), who consider this pattern “benign.”
normal adult EEG; in children and infants, various types of slow patterns appear. In adults with neurodegenerative disease, a posterior alpha rhythm of normal appearance may be replaced by activity in the theta and delta range; a special pattern with rhythmical widespread (but mainly parietotemporal) 5 to 7/sec activity has been individualized recently by Westmoreland and Klass (77), who consider this pattern “benign.”
Voluntary control of the alpha rhythm and the use of alpha feedback methods have been widely discussed topics since the late 1960s. This work was presumably prompted by the observation of well-modulated alpha during meditation practiced by yogis (78,79) or zen buddhists (Hirai, 1968, and Kasamatsu and Hirai, 1966, both articles cited in Ref. 80). Nowlis and Kamiya (81) and Brown (82) associated alpha rhythm enhancement (on a “voluntary” basis) with a pleasant mood. Further work in this field was done by Wallace (83), Wallace et al. (84), and Banquet (85), who studied states of transcendental meditation. Alpha amplitudes increased or decreased; in some subjects there were periods of low-voltage theta activity. Knott (67) feels that such states are in essence periods of drowsiness and doubts the validity of the view that the high alpha state is a desirable condition. According to Stigsby et al. (86), there is no consistent EEG pattern associated with successful or unsuccessful transcendental meditation. In the end, the alpha rhythm as studied here may simply reflect the level of vigilance (see Ref. 87). Because a relaxed waking state is the optimal condition for the posterior alpha rhythm, however, it is hence reasonable to assume that emotional tension attenuates or blocks the alpha rhythm. This seems to hold true for the state of emotional tension in patients or subjects with pending litigation, after a head injury, or after other forms of physical damage, for example (88).
Cerebral Generators of the Alpha Rhythm
The alpha rhythm is of cortical origin, but the theory of a thalamic pacemaker function has frequently surfaced since the work of Berger (40), who presumed cortical genesis but thalamic governance of the alpha phenomenon. Bishop (89) proposed the concept of corticothalamic reverberating circuits, and Andersen and Andersson (90) are the proponents of a thalamic theory that is based on presumed similarities between human alpha rhythm and experimental barbiturate spindle activity in animals. According to this theory, the alpha rhythm is driven by presynaptic input to cortical neurons from the thalamic level (also see Refs. 91 and 92). This concept has been challenged by Lopes da Silva et al. (39,93). Watanabe (94) has postulated a “somewhat loose but stable oscillator system” subserving the generation of alpha rhythm.
Adrian and Yamagiwa (95) regarded the alpha rhythm as cortical with maximal involvement of the visual area. Important new vistas were opened with the demonstration of some degree of interhemispheric asynchrony between alpha waves (96). It has been presumed that there is more than one alpha generator within the posterior regions of the cerebrum (97); this was further substantiated by depth EEG studies in the human (98). The technique of chronotopography has added further insight into the possibility of multiple sources of alpha generation (99). Further work on interhemispheric phase differences between alpha waves has been carried out with toposcopic analysis (100) and cross-correlation technique (101).
Posterior alpha generation and spread in posteroanterior direction (97) is generally acknowledged, but has been challenged by Inouye et al. (102), who feel that alpha spread occurs in an anteroposterior direction in the dominant as well as in the nondominant hemisphere, based on the method of entropy analysis.
Our comprehension of alpha-rhythm genesis has not strikingly increased in past several decades. It may be assumed that there are corticocortical and thalamocortical systems that interact in the generation of cortical alpha rhythms (103). From the experimenter’s as well as from the clinical electroencephalographer’s viewpoint, there is good reason to presume that alpha rhythm is most definitely a cortical phenomenon but there has been, thus far, no evidence of a synchronizing mechanism at the cortical level. No single neurophysiologic alpha rhythm theory has yet found general acceptance, and there are still uncertainties about the origin and psychophysiologic significance of this remarkable phenomenon. And yet, our insights into the nature of the alpha rhythm (and other EEG rhythms) have been deepening.
Mu Rhythm
Rolandic (central) mu rhythm is in frequency and amplitude related to the posterior alpha rhythm, but its topography and physiologic significance are quite different (Fig. 11.3). Historically, the existence of a special central rhythm was presumed by Jasper and Andrews (2) (“precentral alpha rhythm”), Maddocks et al. (104) (“alphoid activity”), and Schütz and Müller (105) (“high voltage rolandic alpha”). The features of mu rhythm were first described in detail by Gastaut et al. (106) and Gastaut (107), who also included electrocorticographic tracings; these authors introduced the term rhythme rolandique en arceau. The epithet en arceau alludes to the arch-shaped wave morphology, which has also prompted the term wicket rhythm (108). Magnus (109) used the term central alpha (see also Ref. 2). Other terms are arcade rhythm (110), comb rhythm (27), and somatosensory alpha rhythm (111).
Mu rhythm is not detectable in every mature subject; its prevalence is limited unless the “hidden” mu rhythm is visualized with special methods. Mu stands for motor; this rhythm is strongly related to functions of the motor cortex, but the contribution of the adjacent somatosensory cortex must not be ignored.
Mu rhythm and associated beta activity over the sensorimotor cortex have become a topic of special interest in the past two decades. Indeed, mu rhythm as an interesting clinical EEG phenomenon has transcended into a powerful contributor to the understanding of motor activity in general. This development started with the work of Pfurtscheller on event-related desynchronization (ERD) (112,113). The ERD pertains even more strongly to the rolandic mu rhythm (and furthermore to various cognitive tasks and their cortical EEG accompaniment) (114, 115, 116, 117, 118 and 119). In other words, the ERD has become a new criterion
for the assessment of cortical functioning, not only over the motor cortex but also over various cortical regions of strong afferent input related to neurocognitive activities. Further detail on the ERD is found in the work of Pfurtscheller and Lopes da Silva (120,121).
for the assessment of cortical functioning, not only over the motor cortex but also over various cortical regions of strong afferent input related to neurocognitive activities. Further detail on the ERD is found in the work of Pfurtscheller and Lopes da Silva (120,121).
Age and Prevalence
Central mu rhythm used to be considered scarce. The introduction of the International Electrode System (10-20 system) has contributed to a much greater awareness of this pattern. The C3 and C4 electrodes are located over the precentral gyrus in an optimal location for picking up central mu rhythm. The use of a different electrode system revealed a prevalence of only 3.2% (122) and 2.9% (123), figures that lie well below those found in other studies. Gastaut et al. (108) found mu rhythm in 10% of their adult patients; Beek (124) observed this rhythm in 13% of a predominantly psychiatric patient population. In 500 essentially healthy young male adults, Gastaut et al. (125,126) found mu rhythm in 14.4%. Dongier and Dongier (127) noted mu rhythm in 18% of a population of neurotic patients. Figures of 12% were reported by Picard et al. (128) and Simonova et al. (129). In the patients of Niedermeyer and Koshino (130), the prevalence of mu rhythm was 8.1% (182 of 2248); broken down into age ranges, there were 9.0% between ages 0 and 10 years, 13.8% between 11 and 20 years, 8.4% between 21 and 40 years, and 4.5% above 41 years. These authors demonstrated rolandic mu rhythm in a 20-month-old child; this was thought to be an exceptionally early manifestation of mu rhythm, although the data of Stroganova et al. (131) demonstrated central mu rhythm (during a state of attention) in the tracing of an 8-month-old baby with a frequency of about 6 to 8.8/sec. The authors speculated that mu rhythm tends to appear before the occipital alpha-equivalent because, unlike visual stimulation, somatosensory stimulation is present within the uterus. With the use of frequency analysis, the prevalence of mu rhythm reaches values close to 100% (132). Familial occurrence of mu rhythm has been reported by Koshino and Isaki (133).
Morphology, Frequency, and Spatial Distribution
Older synonyms such as rhythme en arceau or wicket rhythm pertain to the wave morphology; mu rhythm shows in most instances a sharp (or spiky) negative and a rounded positive phase. There are a few patterns with a monophasic spiky appearance. Posterior alpha rhythm may have a similar configuration: “wicket spikes” have a similar appearance. The same is true for 14 and 6/sec positive spikes, except for the spike positive and rounded negative component. All these patterns show a spatial distribution that is different from the mu rhythm or occurs at levels of vigilance that are usually incompatible with mu rhythm. The amplitudes of mu rhythm are comparable to those of the posterior alpha rhythm.
Mu rhythm usually occurs in short stretches. Persons with pronounced mu rhythm show long trains of mu rhythm. The most common frequency of the mu rhythm is 10/sec. Frequencies may lie below 9/sec and above 11/sec; a mu rhythm of less than 8/sec is probably a mild abnormality. Mu rhythm is often mixed with local activity around 20/sec. According to Storm van Leeuwen et al. (134), mu frequencies are slightly higher than alpha frequencies. The spatial distribution is essentially confined to the precentral-postcentral region; some spread into parietal leads is not uncommon. The C3 and C4 electrodes are mostly involved; occasionally, a vertex (Cz) maximum is noted. A special vertex mu rhythm with special reactivity (lower limb) was described in aged patients by Farnarier et al. (135). When recorded through bone defects, the mu rhythm is more widely distributed. According to Kuhlman (111), the maximum lies over the postcentral rather than the precentral cortex.
The alphoid (around 10/sec) and the fast (around 20/sec) component of the mu rhythm seem to be inseparably intermingled but, with more advanced technology, spatial separation is possible. According to Salmelin and Hari (136,137) the beta component arises from the motor cortex and the alphoid component from the sensory cortex. These magnetoencephalographic findings have been confirmed by Nashmi et al. (138).
In most persons with mu rhythm, the activity is bilateral but tends to shift from side to side. Coherence function studies have shown lack of bilateral coherence of mu rhythm in normal subjects (134). Strictly unilateral mu rhythm must be scrutinized for the possibility of an ipsilateral rolandic disturbance as, for instance, an early stage of parasagittal meningioma, an arteriovenous malformation, or other types of neoplasms (139). In such cases, even the possibility of a contralateral rolandic lesion must be taken into consideration (107,140,141). Above all, the possibility of a local cranial bone defect, surgical or traumatic, must be ruled out; a single burr hole in the rolandic region enables otherwise hidden mu rhythm to become manifest on the scalp (Fig. 11.4). Thus, mu rhythm represents at least a portion of rhythmical activity beneath bone defects (“breach rhythm,” Ref. 142).
Reactivity
Mu rhythm is blocked by movements. These movements may be active (voluntary), passive, or reflexive (106,107,143, 144 and 145). The blocking effect is bilateral but more pronounced on the rolandic region contralateral to the site of movement; the effect appears prior to the onset of muscular contraction (106,107,122,144,145). According to Chatrian et al. (145), there are delays of 50 msec to 7.5 seconds (average, 1.5 seconds) at the onset of the mu blocking effect after the initiation of the spontaneous flexion of the contralateral thumb, with the ipsilateral lagging behind the contralateral response. Gastaut et al. (106) and Ciganek (146) demonstrated a somatotopic distribution of the blocking effect (according to the functional anatomy of the rolandic cortex), but this observation could not be confirmed by Chatrian et al. (145).
With the use of a gamma band (38 to 40/sec) frequency analysis focusing on the event-related synchronization (ERS) of the gamma component, Pfurtscheller et al. (119) beautifully demonstrated the locus for right and left index finger movement, right toes, and the rather broad and bilateral area for tongue movements.
The mu blocking response is likely to be related to the conceptual design of the movement to be executed. Mere thoughts about performing movements and readiness to move block the mu rhythm (106,107,122,143, 144 and 145). Mu blocking responses have also been demonstrated in persons with amputations of extremities; these mental activities concern movement of a phantom limb (122,147).
Light tactile stimuli also produced a mu rhythm blocking effect that is most evident over the contralateral rolandic region (106,107,109,143, 144 and 145). Kuhlman (111) has placed even greater emphasis on the sensory component and regards mu rhythm as the “idling” of the cortical sensory region, in analogy to the alpha rhythm as “idling” of the visual cortex and its vicinity. His work was carried out with power spectral analysis. This technique shows that central mu rhythm is a more common phenomenon than one would expect from the visual analysis of the scalp EEG (also see the power spectra study of Ref. 112). These authors also showed simultaneous posterior alpha desynchronization (112,113). Coherence functions have also been used for the demonstration of rolandic mu rhythm (132,134).
It has been noted that rolandic mu rhythm is enhanced during intermittent photic stimulation (148) and pattern vision (149). The latter observation would contradict the basic law of mu rhythm as a rhythm of immobility, but would be consistent with extraocular movement control being neuroanatomically distinct from the rolandic motor cortex.
Cerebral Generators of the Mu Rhythm
Jasper and Penfield (150) found evidence of a strictly localized beta (around 20/sec) over the human motor cortex with electrocorticographic recording technique in the locally anesthetized patient. This rhythm could be blocked like mu rhythm with movement, especially contralateral movement, and also with thinking about the execution of movement. This rhythm is obviously the cortical equivalent of rolandic mu rhythm on the scalp. The mu waves on the scalp often show some notching or are imbedded in local fast activity, which is also blocked by movement. Gastaut (107) confirmed the cortical precentral fast activity in electrocorticographic tracings. These data conflict with Kuhlman’s (111) observation of a
postcentral maximum of rolandic mu rhythm (scalp recordings with power spectral analysis). On the other hand, Graf et al. (151) demonstrated precentral 8 to 10/sec mu rhythm in the electrocorticogram of an adult epileptic.
postcentral maximum of rolandic mu rhythm (scalp recordings with power spectral analysis). On the other hand, Graf et al. (151) demonstrated precentral 8 to 10/sec mu rhythm in the electrocorticogram of an adult epileptic.
As mentioned earlier, mu rhythm around 10/sec on the scalp and cortical rolandic 20/sec activity appear to be harmonically related; such a relationship, however, does not exist between mu rhythm and rolandic beta activity on the scalp according to Pfurtscheller (114) who used power spectral analysis. Quantitative EEG studies have also established a clean separating line between mu rhythm and posterior alpha rhythm (152).
A study of the functional significance of the mu rhythm from the human cortex was carried out with subdural electrodes by Arroyo et al. (153). This work also showed the relationship of the cortical mu rhythm to the well-known somatotopic arrangement of the sensorimotor cortex.
Three historical theories have governed the theoretical approach to the mu rhythm: (a) the hypothesis of “neuronal hyperexcitability” (“hyperexcitabilité neuronique”), either diffuse or locally restricted to the rolandic cortex (106,107,110,124); (b) the hypothesis of superficial cortical inhibition (“inhibition corticale superficielle”), comparable to aforementioned theories of “cortical idling”; this theory would explain the suppression of mu rhythm with motor activity (154, 155 and 156,147); and (c) the hypothesis of somatosensory “cortical idling,” turning mu rhythm into an afference-dependent phenomenon (111).
Conditioning, Feedback Training, and Relationship to Vigilance
An impressive international study on mu rhythm and the phasic evolution of conditioning was presented by Gastaut et al. (157). Mu rhythm feedback training has been used for various purposes, including the treatment of epileptic seizure disorders (158, 159, 160 and 161). Roldan et al. (162) observed the development of mu rhythm-like activity in the course of Hatha yoga exercises. This activity was called chi rhythm. It was believed to be a specific rolandic pattern of 11 to 17/sec waves and theta frequencies. Covello et al. (163) felt that several entities of mu rhythm exist, even in one subject, and that their underlying physiologic mechanisms may differ. Personal impressions lend some support to this idea. Earlier observations (130) led to the conclusion that even the slightest degree of lessened vigilance (i.e., earliest drowsiness) was incompatible with mu rhythm; this view has been supported by Schoppenhorst et al. (132). However, there have been some personal observations of patients demonstrating their best stretches of central mu rhythm in the light drowsy state after posterior alpha dropout. Yamada and Kooi (164) even described mu rhythm in sleep (stages 1 and 2, as well as rapid eye movement [REM] sleep) in 20 individuals (19 patients with various complaints). The concept of Covello et al. (163) is certainly in need of further study.
Clinical Significance
Pure rolandic mu rhythm as such is categorically within normal limits. In some cases, however, spiky discharges may be present and even single spikes may stand out. Children with benign rolandic epilepsy may gradually develop mu rhythm when rolandic spike activity subsides and the seizures appear to be under control. Interestingly, in some children with benign rolandic epilepsy, cerebral spikes can be blocked by contralateral movements in the same manner as mu rhythm is blocked (165,166). This response is not present in all children with rolandic spikes; this explains negative responses to motor activity reported by Isch-Treussard (167).
As with many other EEG patterns considered to be within the range of normal, mu rhythm has been associated in the historical literature with a predisposition to any number of neurologic and non-neurologic pathologic conditions, including headache, hypertension, hyperthyroid states, psychiatric disorders, and even eczema (108,123,127,154,168), all with varying degrees of correlation and plausibility. It is known that essentially all healthy adolescents and adults harbor central mu rhythm when power spectra and coherence tests are utilized (132). Why then is mu rhythm evident in the conventional EEG in just a minority of persons? It may be that their mu rhythm is more powerful than that of average persons in order to be picked up from the scalp. There is no good evidence that this represents a clinically pathologic sign, however.
In cases of strictly unilateral mu rhythm, a careful search for bone defects (burr holes) and/or local pathology is indicated. In the wake of ischemic hemiplegic insults, central mu rhythm may disappear or its reactivity may vanish (169).
The “Third Rhythm” (Independent Temporal Alphoid Rhythm)
Rhythmical activity in the alpha and upper theta range can be picked up from epidural electrodes over the midtemporal region (170, 171, 172, 173 and 174). This rhythm is not detectable in the scalp EEG unless there is a local bone defect in the midtemporal region where it has been termed (together with coexisting central mu rhythm in the vicinity) “breach rhythm” by Cobb et al. (142), who regarded the temporal activity as an abnormal rhythm. There is now good reason to presume that the rhythmical temporal lobe activity, which is clearly independent from the posterior alpha and rolandic mu rhythm, constitutes a physiologic rhythm.
The function of this rhythm is still debatable, however. Its presence as an independent temporal alpha rhythm has been demonstrated with the use of magnetoencephalography in healthy adults by Hari (personal communication, 1990, 1991), who felt that this rhythm is strongly related to the cortical auditory function (“auditory alpha rhythm”). Personal data (170, 171, 172 and 173) do not provide good evidence for a relationship to auditory function. In some individuals, cognitive activities result in blocking responses of the third rhythm. Rhythmical activity in the alpha and upper theta range over the anterior temporal-midtemporal region in conventional scalp EEGs can be found in patients with cerebrovascular problems (175) and other types of temporal lobe changes, but this abnormal rhythm can be differentiated from the physiologic “third rhythm” (173).
Beta Rhythm
The Greek letter beta stands for the frequency band above 13/sec. In customary EEG recording, frequencies above 70/sec are considerably attenuated due to filter effects. This establishes a natural upper limit of discernible EEG activity somewhere between 50 and 100/sec. The upper limit of the beta band is either considered open-ended or defined as 30/sec (with gamma frequencies above). Rhythmical beta activity is encountered chiefly over the frontal and central regions. A central beta rhythm is related to the rolandic mu rhythm and can be blocked by motor activity or tactile stimulation, as described above. The amplitude of beta activity seldom exceeds 30 µV. Beta activity may be locally enhanced over bone defects and shows considerable increase (in quantity and voltage) after the administration of barbiturates, some nonbarbituric sedatives, and minor tranquilizers. With very large amounts and high amplitudes of beta activity, abnormal proportions may be reached; in general, however, fast activity alone is seldom a cogent reason for calling a tracing abnormal.
Historically, the discovery of beta activity is closely linked to the first description of the alpha rhythm by Berger (1). His first report on the human EEG of 1929 contains the following passage: “The electroencephalogram represents a continuous curve with continuous oscillations in which … one can distinguish larger first order waves with an average duration of 90 sigma (msec) and smaller second order waves of an average duration of 35 sigma (msec)” (after Ref. 41). In his second report, Berger (176) pointed out: “For the sake of brevity I shall subsequently designate the waves of first order as alpha waves, the waves of second order as beta waves.” Berger (62) reversed earlier views and pointed out “that the beta waves and not the alpha waves of the EEG are the concomitant phenomena of mental activity.” This concept is now provoking renewed interest.
Prevalence of Beta Activity
Beta activity is found in almost every healthy adult. Simon’s (29) statement that beta frequencies have been found in 22% of normal adults probably applies to more conspicuous forms of beta activity. Depth EEG studies and electrocorticograms show a remarkably large share of the beta band; such recordings have been obtained mostly in epileptics or in patients with neurologic or psychiatric problems. Although obtained in patients and not in healthy persons, such depth records are quite informative concerning the spatial distribution of fast frequencies. Every scalp recording performed over a bone defect gives testimony to the wealth of cortical beta activity.
The prevalence of beta activity in the normal person can also be derived from various studies of normal volunteers (30,177, 178, 179, 180, 181, 182, 183, 184 and 185). The results of these studies have been reviewed by Kuhlo (186) in his thorough EEG handbook article on beta rhythms. Later work has been presented by Fortuin and Künkel (187) and Kozelak and Pedley (188).
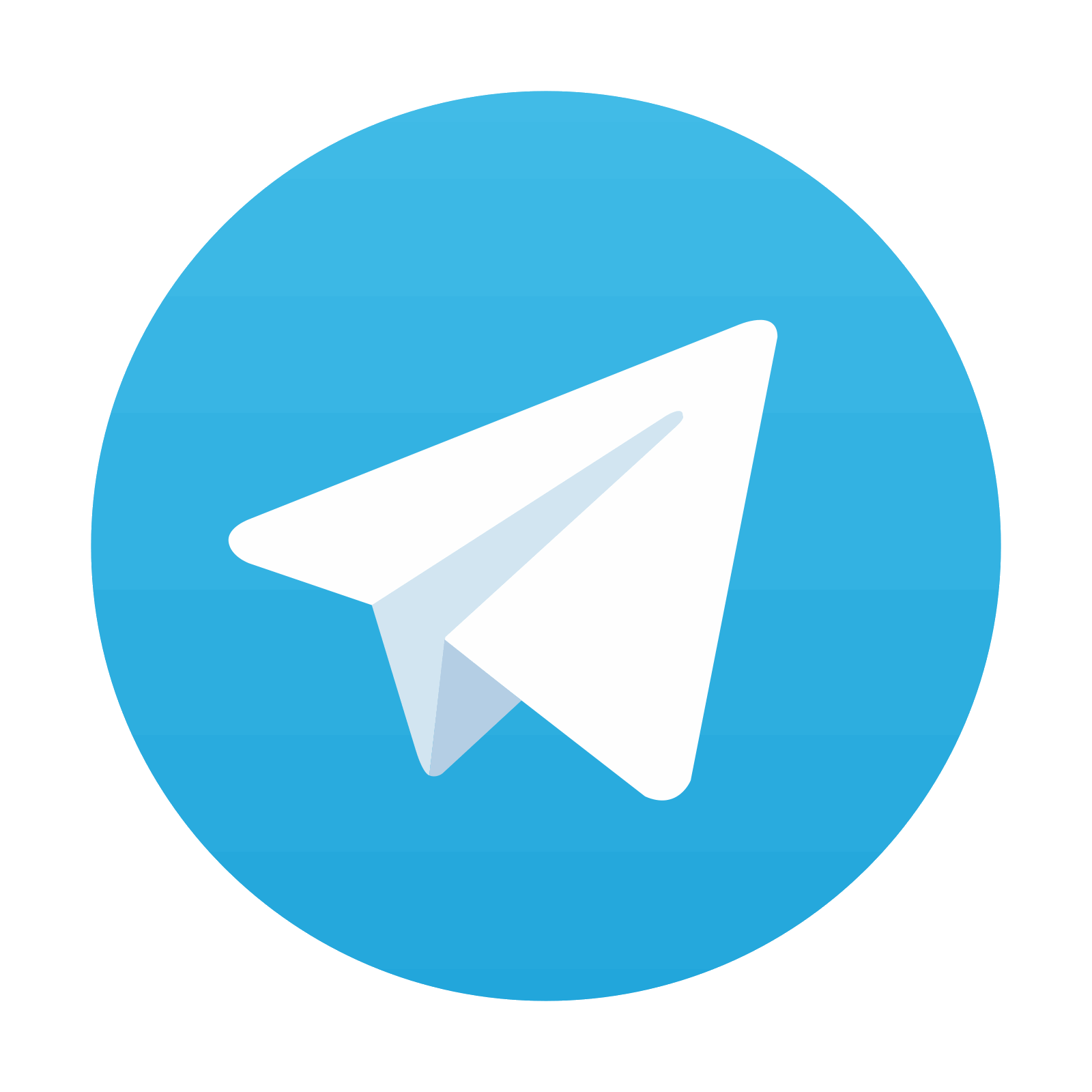
Stay updated, free articles. Join our Telegram channel
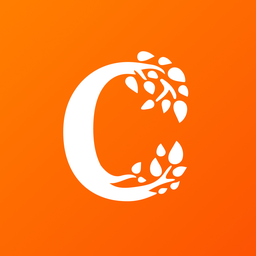
Full access? Get Clinical Tree
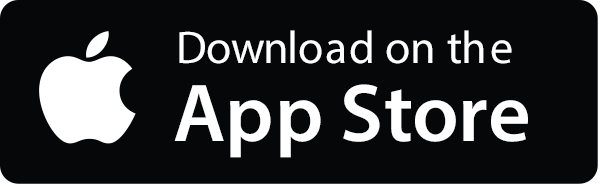
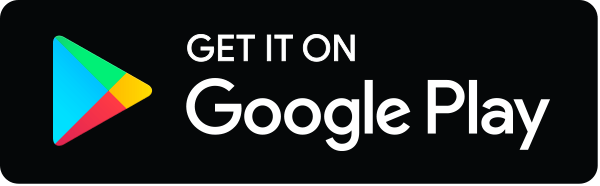
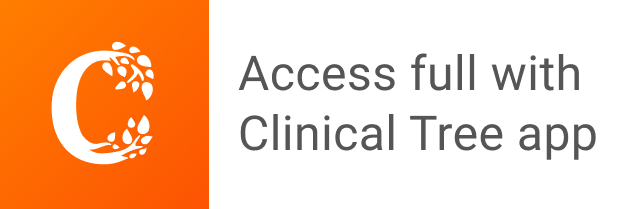