Fig. 4.1
Cortical layering process in mouse embryos. Half coronal section through the forebrain of an E14.5 mouse embryo (upper left scheme). Successive waves of post-mitotic projection neurons are generated between E11 and E18 in the dorsal telencephalon (cortical interneurons are not represented). A magnified area of the neocortex (boxed with a green square on the coronal section) shows the sequential establishment of the adjacent cortical layers (see timeline). The first wave of projection neurons splits the preplate (PP) into a subplate (SP) and a marginal zone (MZ), which also includes migrating Cajal-Retzius cells (CR). These cells release the glycoprotein Reelin that serves as migration signal. Projection neurons arise from committed progenitors located in the ventricular (VZ) and subventricular (SVZ) zones and migrate radially towards the cortical plate (CP) by locomotion on radial glia processes (black lines). At birth, the neocortex is composed of six molecularly distinct (different colours) layers (I–IV) established in an inside-out fashion above the white matter (WM)
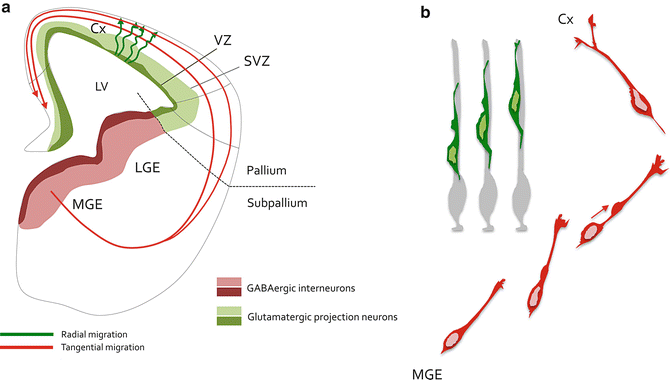
Fig. 4.2
Neuronal migration in the developing cerebral cortex. (a) Scheme representing a hemisection of an E14.5 mouse embryo forebrain. Glutamatergic projection neurons are born around the lateral ventricle (LV) from stem and cortical progenitors residing in the pallial ventricular (VZ, dark green) and subventricular (SVZ, light green) zones. Projection neurons migrate radially (green tortuous arrows) to integrate the cortical plate of the cortex (Cx). Cortical interneurons are GABAergic and arise from the subpallial medial (MGE) and caudal (CGE) ganglionic eminences. They are born from stem and progenitor cells located in the VZ (dark red) and SVZ (light red). These neurons undergo radial migration along various paths (red) to integrate the developing cortical wall. (b) Morphological remodelling of cortical neurons during migration. Radial migration of projection neurons (green) is a directed movement characterized by locomotion on radial glia guides (light grey). These neurons are bipolar during locomotion and undergo short-distance nucleokinesis and limited remodelling of the leading process. On the other hand, cortical interneurons (red) navigate in the forebrain parenchyma through a migration process characterized by an extensive nucleokinesis (red arrow) that comes together with a highly dynamic growth cone, which give rise to temporary branches that are both requires for proper migration
First identified as cell cycle inhibitors, mediating the growth inhibitory cues of upstream signalling pathways, the cyclin-CDK inhibitors of the Cip/Kip family composed of p21Cip1, p27Kip1, and p57Kip2 have emerged as multifunctional proteins with roles extending beyond cell cycle regulation. Cip/Kip proteins in general, and p27Kip1 (renamed p27 further in the text) in particular regulate cell migration in various tissues in physiological or pathological conditions (Baldassarre et al. 2005; Besson et al. 2004b; Itoh et al. 2007; Kawauchi et al. 2006; McAllister et al. 2003; Nguyen et al. 2006). In this chapter, we discuss how p27 acts as a modular protein to coordinate critical steps of neuronal migration in the developing cerebral cortex.
2 Old Players, New Functions –Revisiting the Role of Core Cell Cycle Regulators in Cerebral Cortical Neurogenesis
The generation of cortical neurons by progenitor cells is a finely tuned process that requires a tight coordination of multiple cellular activities, including cell specification, cell cycle exit, cell migration and neuronal differentiation. Achievement of these multiple biological steps relies on implementation of specific genetic inputs as well as on molecular signaling pathways triggered by specific extracellular cues, including growth factors and neurotransmitters (Heng et al. 2007; Nguyen et al. 2001). However, the mechanisms that integrate these cellular processes into appropriate developmental programs remain poorly understood. Accumulating evidence supports the existence of a complex relationship between cell cycle components and factors promoting embryonic development in various animal models (Godin et al. 2012; Nguyen et al. 2006; Ohnuma et al. 1999; Reynaud et al. 2000; Vernon et al. 2003; Joseph et al. 2009). More specifically, Cip/Kip proteins are expressed in both, cycling progenitors and postmitotic neurons of the mouse developing cerebral cortex where they modulate neural specification and migration in addition to their “first-identified” function as cell cycle regulators (Itoh et al. 2007; Kawauchi et al. 2006; Nguyen et al. 2006; Tury et al. 2011). Our work revealed that the most abundant Cip/Kip in the embryonic telencephalon is p27, which promotes projection neurons specification through stabilization and thus progressive accumulation of the basic helix-loop-helix (bHLH) proneural transcription factor neurogenin 2 (Ngn2) in dorsal telencephalic progenitors (Nguyen et al. 2006). In addition, p27 acts as key cellular cytoskeleton modulator, thus promoting specific migratory steps of both cortical projection neurons and interneurons (Godin et al. 2012; Nguyen et al. 2006). Later during adulthood, the brain retains stem/progenitor cells in the subventricular zone (SVZ) of the lateral ventricles and in the subgranular zone (SGZ) of the dentate gyrus (DG) in the hippocampus that support continuous generation of new neurons (Fig. 4.3). All Cip/Kip proteins are expressed in the postnatal brain where they play central function for the regulation of neurogenesis. Indeed, they are detected in adult neurogenic regions where they control proliferation kinetics (Doetsch et al. 2002; Li et al. 2009) and promote cell cycle exit (Pechnick et al. 2008) of specific progenitor subtypes. In addition, p21 and p57 control the pool of brain neural stem cell (NSC) by contributing to the regulation of their quiescence (Furutachi et al. 2013; Kippin et al. 2005). Interestingly, the number of stem cells increases in selected adult tissues (including the retina and the lung) of a knockin mouse line characterized by expression of a cell cycle dead mutated version of p27 [p27CK-, a mutant that cannot interact with cyclins and CDKs (Besson et al. 2004b); see also Box 4.2]. This suggests that p27 controls stem cell amplification in selected adult tissues (Besson et al. 2007). Although classical function as cell cycle regulators have been attributed to Cip/Kip proteins in the adult brain, there is currently no demonstration of any additional cell cycle unrelated activities in this specific context. This issue should be further explored.
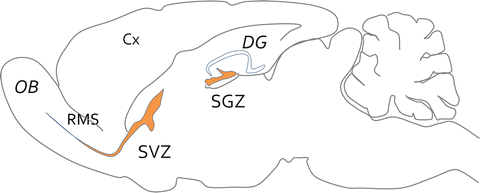
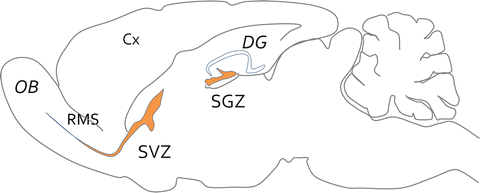
Fig. 4.3
Neurogenic niches of the adult brain. Scheme of a parasagittal section made through the brain of an adult mouse. While no more neurogenesis takes place in the cortex (Cx), two focal brain neurogenic areas (orange) have been clearly identified after birth. The more productive one is the subventricular zone (SVZ) of the lateral ventricle. Adult stem cells are quiescent and upon activation give birth to transient amplifying progenitors that further differentiate into neuroblasts that migrate along the rostral migratory stream (RMS) towards the olfactory bulbs (OB). They differentiate into granular or periglomerular neurons. The second neurogenic niche is the subgranular zone (SGZ) of the dentate gyrus (DG), which give rise to limited number of excitatory granule neurons that settle into the granular layer of the DG
3 Kip Movin’ in the Brain – p27 Promotes Neuron Migration by Regulating Cell Cytoskeleton Dynamics
During migration, neurons undergo major morphological changes (Box4.1) that are accompanied by dramatic shifts in cytoskeleton structure and centrosome positioning. Cell migration is initiated by the protrusion of a leading neurite ending with a structure similar to a growth cone (Tsai and Gleeson 2005). Extension of the highly polarized leading process requires protrusion forces exerted by actin polymerization, microtubule growth and establishment of adhesion complexes that link the extracellular substrate to the actin cytoskeleton (Marin et al. 2006). The centrosome, which comprises the microtubule organisation centre (MTOC), is located ahead of the nucleus in the direction of migration. This elongation step is followed by formation of a swelling, characterized by a cytoplasmic dilatation, that encompass the centrosome, the golgi apparatus, the mitochondria and the endoplasmic reticulum. The nucleus and the centrosome are attached together, through a fork-like structure that enwraps the nucleus in a cage like fashion (Xie et al. 2003). Then, the nucleus relocates into the swelling through nucleokinesis (Schaar and McConnell 2005). Nuclear movement varies depending on the cell type with short and high amplitude for projections neurons and interneurons, respectively. Discrepancies about the contribution of actin and microtubule networks to the forward migration of the nucleus argue for either pulling or pushing forces to generate somal motion (see also Chap. 7).
Box 4.1 Cortical Neurons: Two Distinct Modes of Migration
The two major classes of cortical neurons adopt distinct modes of migration to reach their final position within the cortical plate. Projection neurons undergo directed migration along straight radial glia guides (Gupta et al. 2002). The migration process is divided into four phases (see also Chap. 1): bipolar progenitors migrate through the subventricular zone (SVZ) independently of the radial glia scaffold. When they reach the intermediate zone (IZ), projections neurons stop their migration, sprout multiple neurites and become multipolar (Noctor et al. 2004). Neurons further convert to a bipolar shape, attach to radial glia and move to the cortical plate by glia-guided locomotion. Once connecting to the pia, neurons undergo a final nuclear translocation to settle at appropriate position in the cortical plate (Noctor et al. 2004). Cortical interneurons extend multiple branches and move along various tangential paths that run across different substrates in the telencephalon (Anderson et al. 1997; Tamamaki et al. 1997), including progenitor cells, post-mitotic neurons as well as radial glia fibers (Yokota et al. 2007). Migration of interneurons results from successive cycles of morphological changes that couple saltatory progression of their cell body with dynamic remodelling of their leading process. The nucleus alternates between resting phases that correlate with elongation of the leading process and movement phases associated with splitting of growth cone-like structures that give rise to new branches (Bellion et al. 2005). This stepwise behavior relies on cytoskeletal transformations that promote rostral translocation of a cytoplasmic dilatation encompassing the centrosome and the Golgi apparatus into the extending leading process. This is followed by forward migration of the nucleus, and its perinuclear cytoplasm, a process named nucleokinesis (Marin et al. 2010). Finally, interneurons undergo retraction of the trailing process and branching of the leading process (Bellion et al. 2005).
3.1 p27, an Unexpected Regulator of Cell Migration
The contribution of p27 to cell migration was first reported in hepatocellular carcinoma cells: transduction of a TAT-p27 protein promoted their migration in vitro (Nagahara et al. 1998). Further studies confirmed that p27 acts as a regulator of cell migration in a variety of cell types, including fibroblasts, vascular smooth muscle cells, endothelial cells, and cortical neurons (Diez-Juan and Andres 2003; McAllister et al. 2003; Sun et al. 2001; Godin et al. 2012; Nguyen et al. 2006) (Fig. 4.4). Besson and collaborators showed that p27 null mouse embryonic fibroblasts (MEFs) have reduced motility compared to wild type MEFs and re-expression of p27 rescued motility defect of p27 null MEFs (Besson et al. 2004b). Furthermore, re-expression of a p27 mutant that cannot bind cyclins and CDKs (p27CK-; see Box 4.2) also restored migration, suggesting that the effect of p27 on cell motility was independent of its cell cycle activity (Besson et al. 2004b). The first demonstration of an in vivo cell cycle-unrelated activity of p27 came from the analysis of neuronal migration during embryonic development. Overexpression of wild-type or p27CK- efficiently promoted migration of projection neurons to the cortical plate, while overexpression of other members of the cip/kip family did not affect radial migration (Nguyen et al. 2006). Indeed lack of p27 impaired: (1) Radial migration of projection neurons: p27 knockdown projections neurons remained in subventricular and intermediate zones (Nguyen et al. 2006; Kawauchi et al. 2006) and they failed to acquire multipolar morphology in the intermediate zone (Kawauchi et al. 2006); (2) Tangential migration of interneurons: conditional removal of p27 in interneurons led to decreased migration velocity that arose from both nucleokinesis and branching defects (Godin et al. 2012). Lack of p27 expression in cortical interneurons delayed the course of tangential migration rather than permanently blocking it. Radial and tangential migrations in p27CK mice were not affected suggesting that cortical migration properties of p27 are independent of its cell cycle activity (Godin et al. 2012; Nguyen et al. 2006). We will further discuss the mechanisms by which p27 regulates migration with an emphasis on cortical neurons.
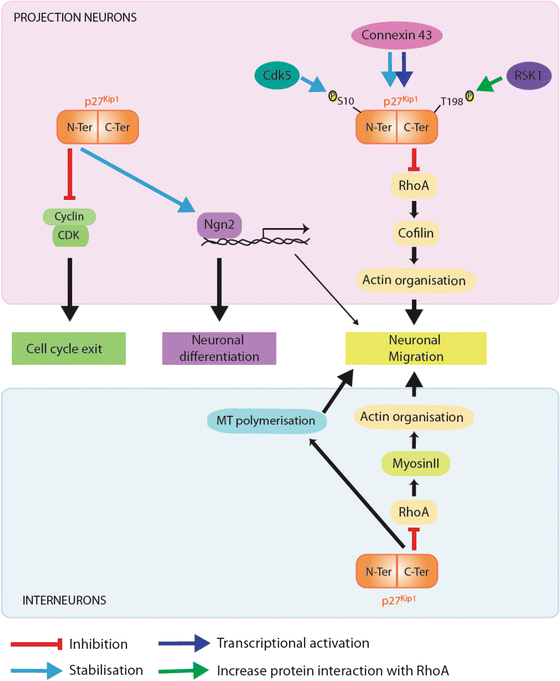
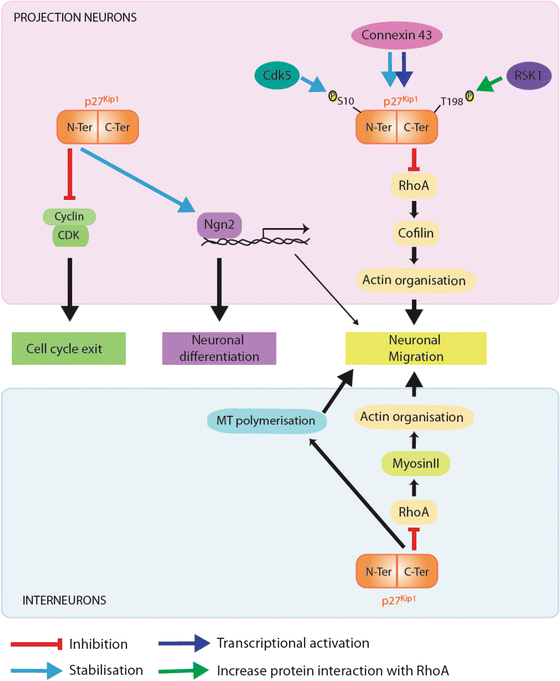
Fig. 4.4
Different roles of p27 during corticogenesis. p27 promotes cell cycle exit by associating with specific Cdk/cylins complexes through a N-terminal binding domain and hence blocking their catalytic activity and preventing G1-S phase transition (molecular pathway in green). By stabilising Ngn2 in the nucleus of cortical progenitors, p27 regulates neuronal differentiation, an activity that resides in its N-terminal half (molecular pathway in purple). p27 promotes the migration of both types of cortical neurons (projection neurons, upper panel; cortical interneurons, lower panel) by blocking RhoA signalling pathway, an activity residing in its C-terminal domain (molecular pathway in yellow). While p27 regulates actin cytoskeleton through activation of cofilin, an actin depolymerizing factor in projection neurons, it controls actomyosin-contractions by fine-tuning myosin II activity in cortical interneurons. In cortical interneurons, p27 additionally controls migration through regulation of MT dynamics (pathway in blue). p27 could also indirectly promote migration through Ngn2 inducing the transcription of target genes which regulate radial migration. Phosphorylation of p27 at Serine 10 (Ser10) by cdk5 regulates its stability and cytoplasmic localization. Phosphorylation at threonine 198 enhances p27 interaction with RhoA (green arrow). Finally connexin 43 acts as an upstream regulator of p27 by controlling both synthesis (dark blue arrow) and stability (light blue arrow)
Box 4.2 Major Domains and Post-translational Sites of p27 Protein
A detailed examination of the p27 protein identifies two major regions, the N-terminal, which according to homology with p21 and p57 showed the capability of inhibiting the kinase activity of cyclin-CDK complexes, and a C-terminal region that interacts with several proteins involved in processes not correlated to the cell cycle control. p27 interacts with cyclins and CDK through two distinct domains (30/32 and 62/64; Box 4.2). N-terminal half, but not Cyclin-CDK interactions domains, is required for p27-dependent stabilisation of Neurogenin2 (Ngn2) protein in cortical progenitors, probably through interaction domains with specific ubiquitin ligases that may target Ngn2 to the proteasome for degradation (Nguyen et al. 2006). When localized at cytosolic cellular compartment, p27 interacts with various proteins including RhoA (Red box, 190–198) (Godin et al. 2012), Rac, stathmin (dashed box, 170–198) (Baldassarre et al. 2005), Grb2 (90–96) (Kardinal et al. 2006) and 14-3-3 (Fujita et al. 2002). While both halves of p27 protein binds to microtubule in vitro, proline-enriched domain located in the C-terminal region (green box) is crucial for its microtubule-associated function (see further in the text) (Godin et al. 2012). Shuttling between nucleus and cytoplasm is crucial for regulation of p27 functions. A bipartite nuclear localization signal (NLS, 152/153-166/168), which is recognized by the alpha/beta importins, allowing p27 transport into the nucleus (Sekimoto et al. 2004; Zeng et al. 2000) and a putative nuclear export signal (NES, 32–45) have been identified. Finally, p27 post-translational modifications may also control its function, by regulating subcellular localisation (pS10, pY74, pY88, pY89) (Viglietto et al. 2002; Sekimoto et al. 2004; Liang et al. 2002; Kardinal et al. 2006; Ishida et al. 2002; Fujita et al. 2002, 2003), stability (pS10, pT187, AcK100) (Tsvetkov et al. 1999; Perez-Luna et al. 2012; Ishida et al. 2000) or protein-protein interaction (pT198) (Larrea et al. 2008; Fujita et al. 2003). Phosphorylation sites that may regulate p27 migratory activities are highlighted in blue.


3.2 p27 and Actomyosin-Based Cytoskeleton
The first understanding of the mechanisms by which p27 regulates cell migration came from studies performed with p27 −/− mouse embryonic fibroblasts. They revealed that p27 promotes migration by inhibiting the Rho-kinase pathway (Box 4.3) (Besson et al. 2004b). Motility of p27 −/− mouse fibroblasts was impaired as a result of increased RhoA activity. Basal migration level could further be restored by inhibiting the Rho-kinase ROCK (Besson et al. 2004b). Indeed, p27 −/− cells had increased numbers of stress fibers and focal adhesions as well as elevated levels of Rho-GTP (Besson et al. 2004b). Overexpression experiments showed that p27 interacts with RhoA, thereby preventing RhoA activation by interfering with RhoA binding to its GEFs, but not to its effectors (Besson et al. 2004b). It’s worth noting that modulation of p27 expression level can lead to distinct migratory responses depending on the cellular context (Besson et al. 2004a).
The regulation of RhoA by p27 is critical for proper migration of both projection neurons and interneurons in the cortex of developing mice (Godin et al. 2012; Nguyen et al. 2006; Kawauchi et al. 2006; Itoh et al. 2007) (Fig. 4.4). Although, inhibition of RhoA signalling by p27 resulted in changes in actin cytoskeleton in neurons, discrepancy between downstream mechanisms have been described in these two neuronal classes. Recent work performed with projection neurons suggested that p27-mediated block of Rho-kinase pathway promoted actin reorganisation by activating cofilin, an actin-severing enzyme. Indeed, knockdown of p27 in cortical projection neurons lead to increased phosphorylation of cofilin through ROCK but not PAK1 signalling pathways (Gungabissoon and Bamburg 2003), suggesting that defect of migration arose from excessive stabilisation of the actin network in p27-deprived projection neurons (Kawauchi et al. 2006). These data were supported by some work performed with fibroblasts showing that p27 suppresses the activity of the RhoA-ROCK (Rho-kinase) pathway, thereby decreasing Ser 3 phosphorylation of cofilin (Besson et al. 2004b). However, the extent to which cofilin mediates p27 activity in neuronal actin cytoskeleton remodelling remains unclear since overexpression of a constitutively active form of cofilin prevents radial migration rather than promoting it (Kawauchi et al. 2006). In addition, the lack of p27 expression in cortical interneurons correlated with partial inactivation of cofilin but without an accumulation of actin microfilaments. This could be explained by the concomitant expression of gelsolin, another actin-severing protein, as detected in cortical interneuron in vivo (Godin et al. 2012). Together, these results suggest that the severing of actin is driven by multiple and redundant mechanisms, as G/F actin ratio remained unchanged in p27 −/− interneurons (Godin et al. 2012) (Fig. 4.4). Indeed the main candidate target of the Rho-kinase pathway in migrating interneurons was myosin II (Fig. 4.4). This protein complex is involved in actomyosin-based contractions and p27 fine-tuned myosin II activity at the rear of the nucleus and within the growth cone. The lack of p27 led to a hyperactivation of myosin II that came together with increased frequency of nucleokinesis as well as excessive branching activity. These cellular defects were rescued by treatment with pharmacological inhibitors of the Rho effector ROCK or of the myosin light chain kinase (MLCK) (Godin et al. 2012). Although nucleokinesis and leading process branching activities were rescued, the elongation of neurites remained affected, suggesting the existence of additional substrate of p27 in migrating interneurons. In addition, targeting The RhoA/myosinII pathway was not sufficient to rescue basal level of velocity (Fig. 4.5).
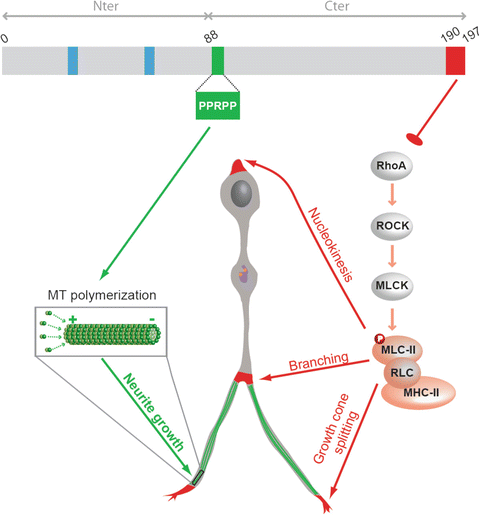
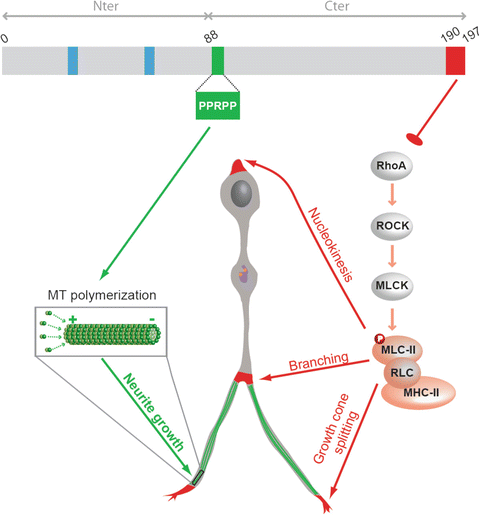
Fig. 4.5
Summary scheme illustrating the molecular pathways by which p27 regulates cortical interneurons tangential migration. p27 acts as a master regulator of cytoskeletal transformations to control cortical interneuron migration in vivo. p27 controls neurite extension and nucleokinesis through independent pathways. p27 controls nucleokinesis, branching and growth cone splitting through inhibition of RhoA and regulation of acto-myosin contractility (molecular pathway in red). p27 promotes neurites extension by regulation microtubule polymerisation (molecular pathway in green). p27 expression rescued tangential migration defects in brain slices from p27-null embryos, including numbers of interneurons that reached the cortex and mean interneuron velocity in knockout embryonic slices. However, a p27 mutant (p27190-4A) that lacks the ability to regulate both RhoA signalling (p27-190) and polymerisation of MTs (p274A) could not rescue interneuron migration, while single mutants only partially contributed to this process. This suggests that both novel cell cycle-unrelated activities are required to support proper tangential migration of interneurons in the cerebral cortex. Abbreviations, MLC-II for myosin light chain II, RLC for regulatory light chain, MHC for myosin heavy chain II. Blue boxes: cyclin and CDK interacting domain; orange dots: centrioles; purple line: golgi apparatus (Adapted from Godin et al. 2012)
Altogether, these data demonstrate that p27 controls Rho-kinase/myosin II activity to fine-tune actomyosin-based contractions that take place behind the nucleus during nucleokinesis and the leading process growth cone. However, there is an apparent discrepancy between the wide cytoplasmic distribution of p27 and the discrete location of actomyosin activity in migrating interneurons. Several works showed that p27 undergo multiple and dynamic post-translational modifications among which some are important to regulate its nucleo-cytoplasmic shuttling (Viglietto et al. 2002; Sekimoto et al. 2004; Liang et al. 2002; Kardinal et al. 2006; Ishida et al. 2002; Fujita et al. 2002, 2003; Zhang et al. 2013; Connor et al. 2003). Such modifications may indeed control the specific and dynamic accumulation of a pool of p27 at the rear of the nucleus and in the growth cone of actively migrating interneuron. This hypothesis should be further investigated.
Box 4.3 RhoA, a Master Regulator of Actin Dynamics
The Rho family of GTPases, which includes Rho, Rac and Cdc42, regulate cell morphology, cytokinesis and cell motility through reorganization of actin filaments (Bar-Sagi and Hall 2000). RhoA activation promotes actin stress fiber formation, focal-adhesion assembly, as well as actin-myosin contractility (Etienne-Manneville and Hall 2002). The RhoA pathway leads to a decrease in stress fibers and focal adhesions, which increases cell motility. RhoA exists in a GDP-bound inactive state and a GTP-bound active state. Guanine nucleotide exchange factors (GEFs) catalyse the release of GDP, allowing GTP to bind RhoA, leading to activation of its downstream effectors; the Rho-kinases, ROCK1 and ROCK2. Stress fiber formation and focal-adhesion assembly and stability are mediated by the activation of LIM domain-containing protein kinase (LIMK) by ROCK, which in turn phosphorylates and inhibits cofilin, an actin depolymerization factor. ROCK also controls actomyosin contractility, by directly phosphorylating the light chain of myosin II (MLC-II) (Amano et al. 1997) or indirectly via inhibition of the MLC phosphatase MLCP (Kimura et al. 1996) or activation of the MLC kinase MLCK (Chrzanowska-Wodnicka and Burridge 1996). Importantly, myosin activation promotes cell migration by driving translocation of the cell body and retraction of the rear of the cell during migration (Webb et al. 2002).
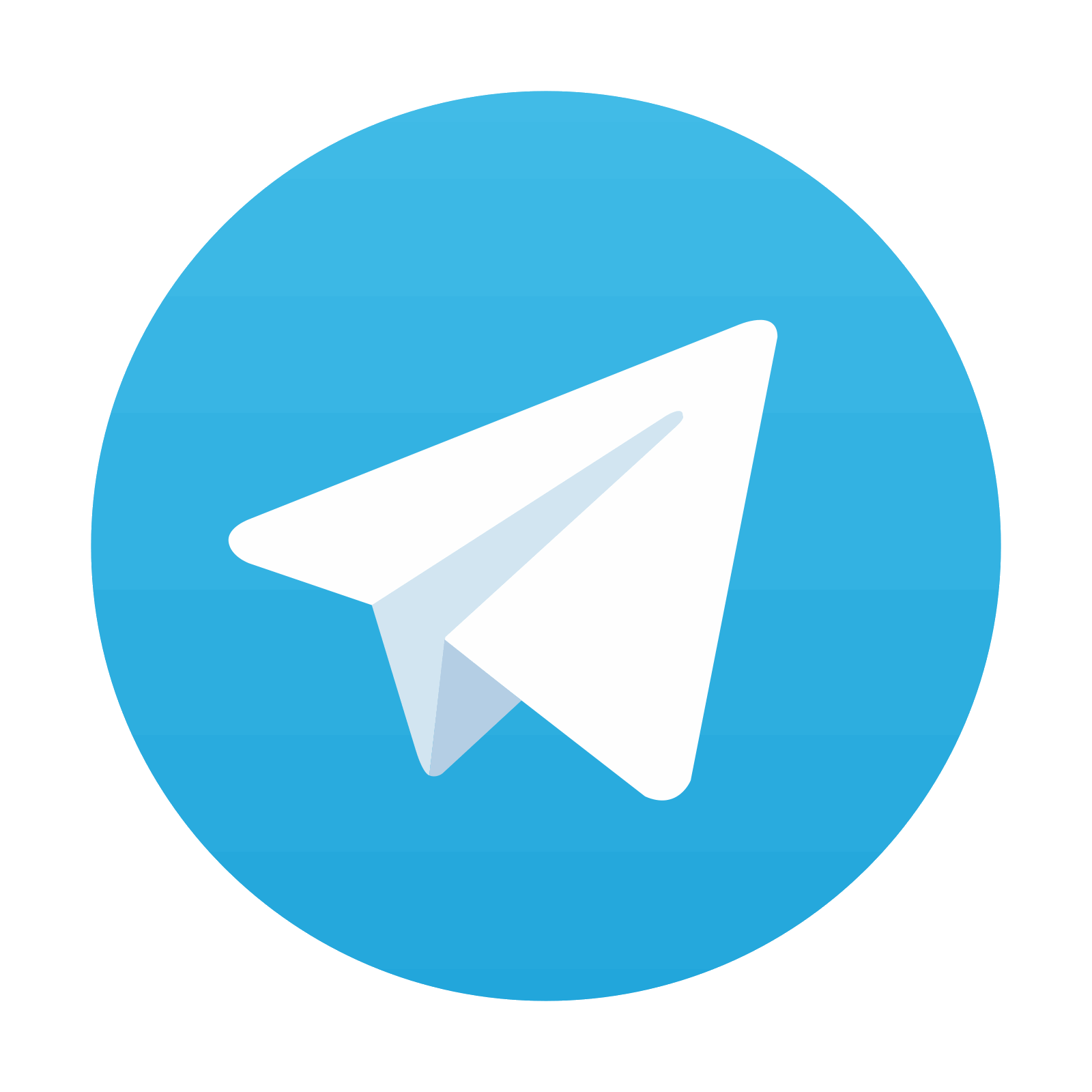
Stay updated, free articles. Join our Telegram channel
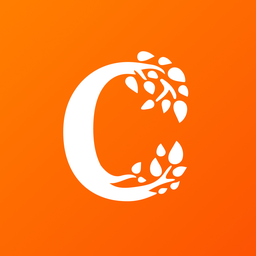
Full access? Get Clinical Tree
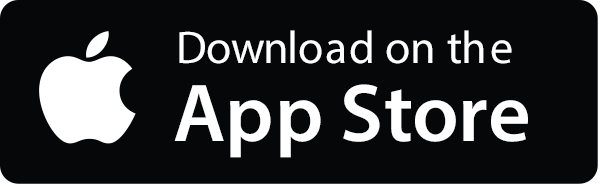
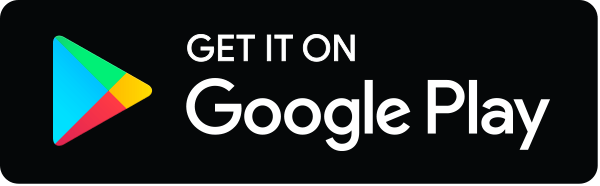