Introduction
Computed tomography (CT) and magnetic resonance imaging (MRI) provide structural images of the brain. CT allows early diagnosis of surgically treatable intracranial space-occupying lesions in traumatic brain injury (TBI) and intracranial hemorrhage and exclusion of intracranial hemorrhage before thrombolysis. MRI is very sensitive at detecting white matter abnormalities, and provides early diagnostic and prognostic information in stroke and TBI. However, therapeutic strategies based solely on structural images are limited because structural changes are late and irreversible. Moreover, conventional imaging is poor at assessing functional recovery. Functional imaging allows early documentation of reversible ischemia in vivo, validation and calibration of bedside clinical monitoring tools, characterization of pathophysiology in individual patients, and early outcome prediction.
Functional CT and MRI Versus Radioligand Techniques for Imaging Physiology
Functional imaging approaches in clinical and experimental stroke have traditionally used cerebral blood flow (CBF) thresholds for ischemia and have succeeded in identifying useful predictive values for tissue survival or death.
Although fewer studies are available in the context of head injury, similar approaches now attempt to identify critical physiologic thresholds for tissue survival in TBI. Xenon CT can provide absolute quantification of CBF, but this technique does not provide whole brain coverage, with imaging typically being limited to two to four selected slices. Although absolute quantification of physiologic parameters allows the most rational application of these thresholds to clinical contexts, clinically useful data can be obtained from assessment of relative changes in cerebrovascular physiology. Perfusion CT or MRI with intravenous contrast media allows estimation of relative CBF and volume (rCBF and rCBV, respectively). At a qualitative level, functional MRI (fMRI) using blood oxygen level dependent (BOLD) contrast allows identification of regional brain activity and the assessment of functional connectivity, but no direct measure of cerebrovascular adequacy. Proton magnetic resonance spectroscopy ( H-MRS) studies show changes in absolute or relative levels of metabolites, and so can document the consequences of ischemia. Lactate increases and N-acetyl aspartate (NAA) reductions in normal-appearing tissue in head injury probably represent ischemia and neuronal loss, respectively, although acute, reversible NAA reductions may represent mitochondrial dysfunction. Changes in choline, creatine, and myoinositol also have been demonstrated, but their significance is less certain. NAA reductions appear to be an important marker of poor outcome, but their potential reversibility implies that late studies may be required to define long-term significance. In addition, although some high-resolution studies have been published, current implementation of the technique is generally limited by relatively poor spatial resolution, limited brain coverage, and long imaging times.
The major advantage of radioligand techniques such as positron emission tomography (PET) and single photon emission computed tomography (SPECT) is the opportunity to specifically aim at a target pathophysiologic process, and especially with PET, the high sensitivity to detect physiologic changes in brain physiology. To date, PET is probably the most selective and sensitive method to visualize and measure in vivo receptor density and oxidative metabolism. However, unlike MRI, the main limitation of radioligand techniques is the exposure to radiation. For PET and SPECT, the radiation exposure typically ranges between 2 and 10 mSv (depending on the specific radioligand use), corresponding to between one and four times annual natural background. Another limitation is the importance of partial volume effect for small structure that limits the spatial resolution to 3 to 5 mm for PET and to 10 mm for SPECT. Moreover, the short half-life of positron emitters used for PET as 11 C or 15 O requires the presence of a cyclotron in the site where it is used: this increases costs.
Methodologic Issues
In both PET and SPECT, a radioactive isotope is administered and external detectors measure brain radioactivity. To avoid prolonged radioactive exposure, relatively short-lived isotopes are used to image cerebral physiology and metabolism or to label proteins, receptors, or neurotransmitters ( Table 29.1 ). The synthesized labeled compound must have the same metabolic or pharmacokinetic proprieties as the unlabeled compound. However, they typically are administered in such small amounts that pharmacodynamic effects are not important. Isotopes of universal atoms such as carbon or oxygen can be used to label molecules without inducing any modification in the chemical structure. Importantly most PET and SPECT studies are performed away from the neurocritical care unit (NCCU), and so careful management and anesthetic technique if necessary are required because the study environment can be unfamiliar.
Methods | Atoms | Isotopes | Half-life for Emission | Examples of Labeled Tracer | Applications |
---|---|---|---|---|---|
PET | Carbon | 11 C | 20.3 min | 11 C-radopride | D2 dopamine receptors |
11 C-flumazenil | GABA-A receptor | ||||
11 C-PK11195 | Activated microglia (brain inflammation) | ||||
11 C-PiB or 11 C-SB13 | Amyloid plaques | ||||
Oxygen | 15 O | 2.1 min | H 2 15 O, C 15 O, and 15 O 2 | CBF, CBV, OEF and CMRO 2 | |
Fluorine | 18 F | 109 min | 18 Fluorodeoxyglucose (FDG) | Glucose metabolism | |
18 Fluoromisonidazole | Tissue hypoxia | ||||
SPECT | Technetium | Tc99m | 6.01 hr | 99m Tc-hexamethylpropyleneamine oxide | CBF |
Iodine | 123 I | 13.1 hr | Iodoamphetamine | CBF |
Positron Emission Tomography
PET is a technique that measures the accumulation of positron-emitting radioisotopes within a three-dimensional object. The production of positron-emitting tracer requires a cyclotron. The positrons that are emitted are the antimatter equivalent of electrons. The collision of an electron and a positron annihilates both particles, resulting in a release of energy in the form of two photons (gamma rays) released at an angle of 180 degrees to each other ( Fig. 29.1 ). This annihilation energy can be detected externally using coincidence detectors, and the locus of each reaction localized within the object by computer algorithms. The tracer distributes to different compartments, according to its pharmacokinetic proprieties. Kinetic modeling that often requires measurement of radioactivity input in plasma is used to derive quantitative measures of the cerebral concentration. Typically this process requires placement of an arterial catheter and rapid sampling of arterial blood during the tracer injection and equilibration, so as to provide an input function for modeling tracer distribution. With some tracers, however, an alternative approach makes use of the inhomogeneous distribution of the tracer in the brain. This property can be used to define a brain area without specific tracer binding, which can be used as a reference area to provide a tissue input function. For example C-flumazenil PET can be used to estimate central benzodiazepine receptor gamma-aminobutyric acid (GABA)-A density, with the pons as a tissue reference without specific binding; this avoids the need to sample arterial blood.

Although PET often is referred to as the gold standard in physiologic or metabolic imaging, the absolute values that it produces result from complex detection and computing systems, both of which are prone to error ( Fig. 29.2 ). Perhaps most important, the accuracy of figures derived from PET is crucially dependent on the validity of kinetic models, many of which have not been tested in the injured brain. Although the data obtained using the technique are extremely valuable, and probably not obtainable by other methods, it is essential that the physiologic insights provided by the PET are assessed critically in the context of these considerations. Although PET clearly can define many complex aspects of cerebral physiology and pathophysiology, it is a research tool that is relatively expensive and not universally available.

Single Photon Emission Computed Tomography
SPECT relies on the imaging of gamma-emitting tracers in which a single photon is emitted (see Fig. 29.2 ).Tc99m-hexamethylpropyleneamine oxide (HMPAO) and 123 I-amphetamine have been used to map CBF. SPECT does not require a cyclotron to be produced. SPECT is a relatively simple and inexpensive technique that can be used to assess three-dimensional cerebral perfusion. However, the images produced are of relatively low resolution and generally nonquantitative.
Although recent papers have reported on quantitative implementations of SPECT, this requires further validation. One interesting development has been the use of analysis techniques such as statistical parametric mapping, derived from functional brain imaging, to examine changes in patterns of blood flow across populations of patients with head injury.
Imaging of Cerebral Blood Flow and Volume
Patient evaluation in neurocritical care often may be confounded by the use of sedative agents and by the metabolic effects of trauma or injury, which may cause primary reductions in cerebral metabolism. A decrease in CBF that is coupled with the depressed metabolism would not represent ischemia. Under these circumstances the only true measure of the adequacy of CBF is a measurement of the oxygen extraction fraction (OEF). A robust identification of ischemia depends on the identification of areas with increased OEF. Triple oxygen PET (using O-labeled tracers in three separate scans (H 2 15 O, C 15 O, and O 2 ) provides valid quantitative data for CBF, cerebral blood volume (CBV), OEF, and the cerebral metabolic rate of oxygen (CMRO 2 ) ( Fig. 29.3 ).

PET can play an important role in understanding the pathophysiologic interplay among CBF brain cellular function in ischemic stroke, carotid artery disease, vascular dementia, intracerebral hemorrhage, and aneurysmal subarachnoid hemorrhage (SAH). In clinical stroke, PET identifies a core area with very low CBF, CBV, and oxidative metabolism, that corresponds to irreversible damage. The surrounding area with normal or elevated CBV and relative CBF preservation with an increase in OEF is termed the penumbra and represents tissue at risk, that may be rescued by physiologic or pharmacologic intervention. The extent of the penumbra measured with PET has been shown in stroke patients to help predict tissue outcome.
After SAH, PET and SPECT have helped to document the modification of CBF and CBV that may show a significant decrease during vasospasm. However, the sensitivity of Tc99m-HMPAO SPECT to detect symptomatic vasospasm is only 50% and perhaps less in predicting vessel narrowing in some vascular distributions. Newer semiquantitative SPECT studies using Hermes brain registration and analysis software (BRASS) may improve on this but requires further study. PET CBF in symptomatic patients also has been found to be less than that observed in asymptomatic patients. The correlation between metabolic markers of brain ischemia measured by microdialysis and a decrease in PET CBF is not excellent, suggesting other causes for metabolic disorders. Nevertheless, PET or the combination of techniques such as PET, perfusion CT and microdialysis can provide insights into the role of cellular hypoxia after SAH, or the response to therapies such as normal saline boluses to induce hypervolemia or blood transfusion to improve oxygen delivery.
Several groups describe the use of PET and SPECT to map TBI pathophysiology. Perhaps the most interesting of these are studies in the acute phase, which aim to elucidate early pathophysiology. In patients with mild to moderate TBI within the first 3 months after injury, CBF measured by SPECT is reduced by 40% to 70% predominantly in the frontal and temporal lobes, basal ganglia, and thalamus. This reduced CBF was associated with unfavorable outcome and poor performance on neuropsychological testing and had a better relationship with long-term outcome than CT or conventional MRI. At 6 months after TBI the extent of regional brain atrophy correlates best with PET measurements of CMRO 2 and CBF and less so lobar OEF. SPECT-detected improvements in CBF also have been observed to occur when there is cognitive recovery after concussion or can help explain flow patterns associated with cognitive fatigue. However, SPECT as a stand-alone test may not be sufficient to confirm that a head injury did or did not occur.
PET has been used to precisely define the ischemic threshold after TBI. A recent whole brain voxel-based study demonstrated the presence of distributed ischemia within 24 hours of TBI, as defined by an increased OEF. In contrast, previous studies have found low global OEF values after TBI, suggesting that global ischemia is uncommon. These differences may reflect difficulties in demonstrating ischemia at different times after injury, when early compensated ischemia is associated with a high OEF. By contrast, progressive cell death within the voxel results in progressive reductions in OEF that culminate in very low values in voxels where most neurons have died. Averaged CBF in areas that eventually progressed to structural abnormality was significantly reduced (median CBF 16.9 mL/100 g/min) compared with nonlesion areas. These variations may be confounded by spatial averaging of OEF within structurally defined regions or across the whole brain, possibly due to the dilution of small regions with increased OEF by larger regions with normal or low OEF. It also is possible that there are mechanisms of ischemic cell death that are not characterized by OEF increases, such as microvascular ischemia and mitochondrial dysfunction. Indeed hypoperfused pericontusional regions where there is oxygen hypometabolism in the subacute stage after TBI may not always result in tissue necrosis, emphasizing the need to know about CBF and metabolism (and from any monitoring modality) before conclusions about ischemia are made.
Both the extent and duration of ischemia contribute to the evolution of irreversible tissue damage. This time dependence may mean that it is not possible to predict tissue outcome based on measurement of CBF, CMRO 2 , or OEF at a single time point after injury. It also is likely that tissue survival will be better predicted by complex interactions of physiologic variables rather than simple univariate thresholds. Initial multivariate analysis appears to provide better separation of damaged and nonlesion tissue, and further investigation may involve using multivariate statistical analysis or neural network techniques to determine if there are combinations of physiologic variables that better predict tissue outcome. Indeed techniques using multivariate algorithms (using data from acute perfusion-weighted and diffusion-weighted MRI scans), to generate voxel-based maps predicting tissue outcome, have been used with some success in stroke studies.
PET studies can be repeated and used to assess changes in physiology with therapeutic maneuvers, such as osmotherapy, hyperventilation, hyperoxia, transfusion, and CPP augmentation. Triple-oxygen PET has been used to address the metabolic effects of hyperventilation in TBI. Hyperventilation to an arterial carbon dioxide tension (PaCO 2 ) of 25 mm Hg produces some increase in OEF, but does cause a significant reduction in global CMRO 2 or in regions of interest with low baseline CBF values. It is interesting that these studies did not detect regions with extremely high OEF values that would suggest true ischemia, even within 24 hours of head injury. Other groups have used PET to show that moderate reductions in PaCO 2 (to 31 mm Hg) can result in increases in the volume of brain tissue with CBF values that are less than ischemic thresholds (<20 mL/100 g/min). Importantly, the development of these ischemic areas that typically are pericontusional or in white matter may not be detected by reductions in jugular bulb oxygen saturations below commonly accepted thresholds for ischemia (<55%). Subsequent studies have shown that ischemia, defined as cerebral venous oxygen content of less than 3.5 mL per 100 mL (that equates to OEF values of 75%-80%), are common in early head injury and may be observed beyond 24 hours post injury. The volume of brain defined as ischemic using these criteria shows an increase with hyperventilation associated with reduced CMRO 2 in some regions. Interestingly, these OEF increases are not only from reduced oxygen delivery but also associated with increases in oxygen demand from hyperventilation. The burden of early ischemia measured using these techniques correlates well with eventual outcome.
Other studies have correlated PET-derived measures of cerebrovascular physiology with bedside monitoring tools such as microdialysis and brain tissue oxygen ( Fig. 29.4 ). These studies help validate and calibrate bedside monitors, define the limits or reliability of a given technique, and provide insights into pathophysiology.

Blood flow and metabolism vary dramatically across the traumatized brain, and different regions may require different therapeutic approaches. This is difficult to achieve in the clinical environment because medical therapies are “global” in nature. However, it is best to measure the effect of common therapeutic interventions in which benefit is demonstrated to decide about continuing that therapy. A potential advantage of PET and monitoring of brain metabolism may be detection of abnormalities in brain regions without structural change on other imaging modalities.
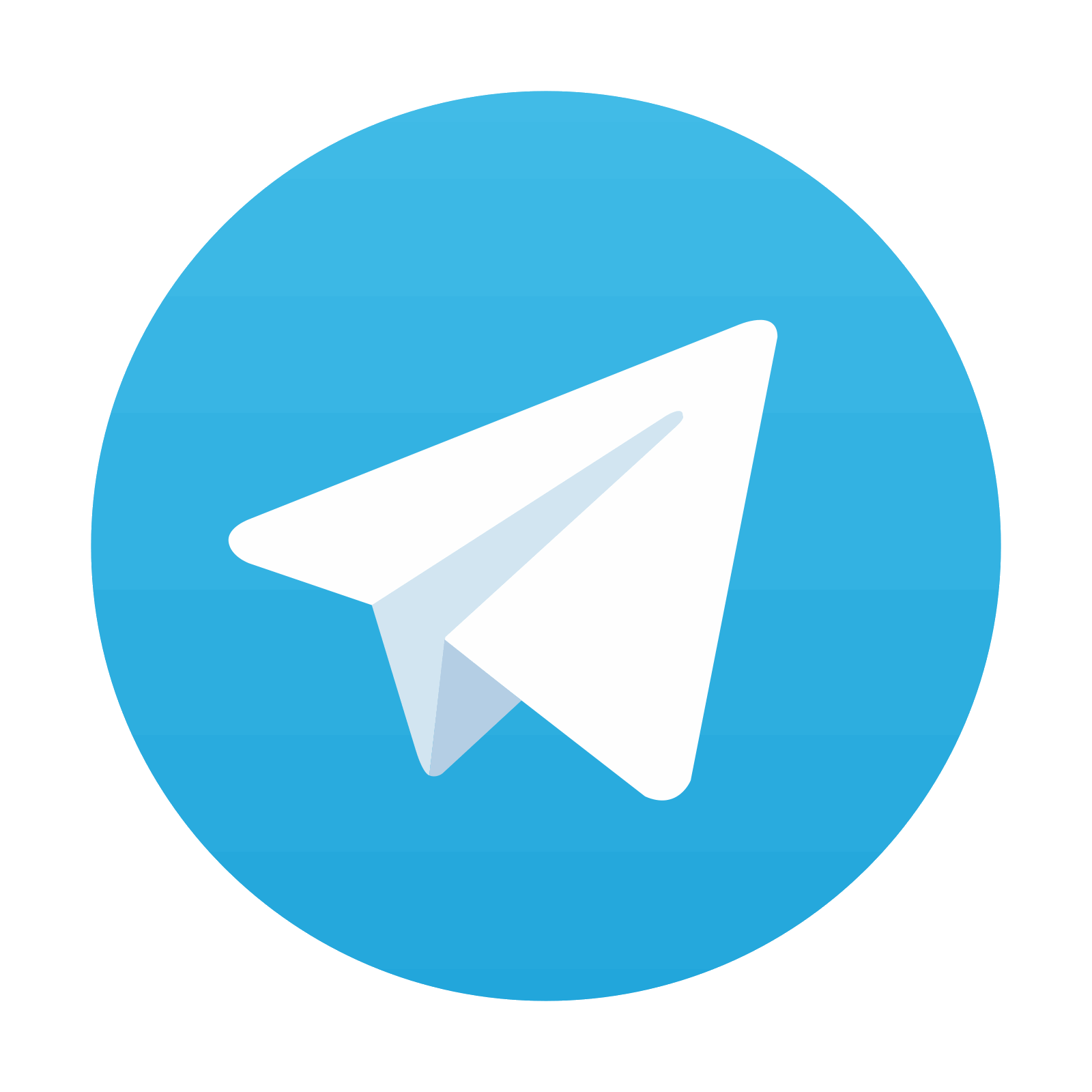
Stay updated, free articles. Join our Telegram channel
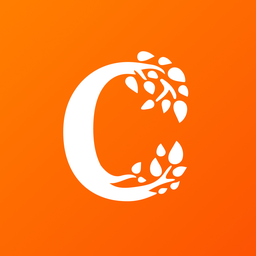
Full access? Get Clinical Tree
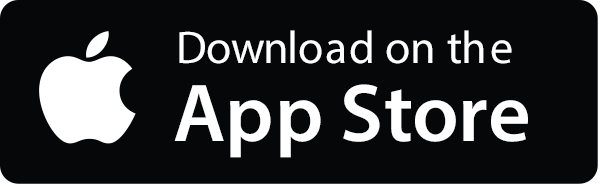
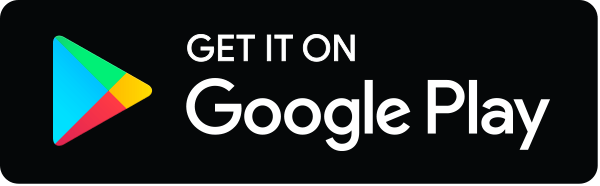