Potassium Channels
Abstract
Astrocytes play a critical role in maintaining extracellular K+ homeostasis. Since neuronal activity is accompanied by efflux of K+ from neurons into the extracellular space, tight regulation of extracellular [K+] is critical to avoid hyperexcitability or synaptic dysfunction. Astrocytes regulate extracellular [K+] by both local K+ uptake and K+ spatial buffering. While there are many astrocytic potassium channels, many studies have implicated the inwardly rectifying potassium channel Kir4.1 as particularly important in setting astrocyte membrane potential and contributing to potassium buffering in the CNS. Alterations in Kir4.1 have been found in both epilepsy and other neurological diseases. Thus, dysregulation of K+ homeostasis may play a key role in the hyperexcitability of epilepsy.
Keywords
Astrocyte; potassium; inward rectification; spatial buffer; reuptake; Kir4.1; KCNJ10
Overview
Astrocytes express various types of ion channels, including K+, Na+, Ca2+, Cl−, and other anion channels and aquaporin water channels (Table 7.1). In this chapter, we focus on the role of astrocyte K+ channels in extracellular K+ homeostasis and alterations in epilepsy. In particular, alterations in the inwardly rectifying potassium channel Kir4.1 have been found in both epilepsy and other neurological diseases [1,2]. Dysregulation of K+ homeostasis may play a key role in the hyperexcitability of epilepsy.
Table 7.1
Ion Channel | Molecular Identity | Localization | Main Function |
Potassium channels | |||
Voltage-independent K+ channels | Two-pore domain K+ channels: | Hippocampus | Contribute to resting membrane potential |
TREK1, TREK2 and TWIK1 | |||
Inward rectifier potassium channels | Kir 4.1 (predominant) | Ubiquitous | Maintenance of resting membrane potential; |
Kir 2.1, 2.2, 2.3 | K+ buffering | ||
Kir 3.1 | |||
Kir 6.1, 6.2 | |||
Outward rectifying K+ channels | |||
Delayed rectifier potassium channels | Kv1.1, Kv1.2, Kv1.5, and Kv1.6 | Ubiquitous | Generally unknown; may be involved in regulation of proliferation |
KD | |||
Rapidly inactivating A-type potassium currents (KA) | Kv1.4 | Hippocampus; astrocytes in vitro | Unknown |
Ca2+-dependent K+ channels | KCa3.1 | Cortex | Unknown |
Sodium channels | Nav1.1, Nav1.2, and Nav1.3 | Spinal cord cultured astrocytes; | Regulation of differentiation, proliferation and migration(?) |
only NAv1.2 was detected in spinal cord astrocytes in situ | Can be upregulated in pathological conditions | ||
Calcium channels | L- (Cav1.2), N- (Cav2.2), P/Q- (Cav2.1), R- (Cav2.3), and T (Cav3.1) | Astrocytes in vitro; functional expression in situ remains controversial | Unknown |
Transient receptor potential, TRP, channels | TRPC1, TRPC4, TRPC5 | Regional distribution is unknown | Store-operated Ca2+ entry |
Chloride channels | CLC1, CLC2, CLC3 | Ubiquitous | Chloride transport; regulation of cell volume |
Volume-regulated anion channels of unknown identity | |||
Aquaporins (water channels) | AQP4 (predominant) | AQP4—ubiquitous | Water transport |
AQP9 | AQP9—astrocytes in brain stem; ependymal cells; tanycytes in hypothalamus and in subfornical organ |
Reproduced with permission from Verkhratsky A, Butt AM. Glial physiology and pathophysiology. Oxford, UK: Wiley-Blackwell; 2013 (Table 4.1).
Astrocyte K+ Channels
Early physiological studies of astrocytes revealed that the resting membrane conductance of astrocytes is dominated by passive K+ currents which maintain the membrane potential (~−80 to −90 mV) close to the potassium Nernst equilibrium potential (EK) [3–6]. Astrocyte K+ channels include voltage-independent K+ channels and voltage-dependent K+ channels (divided into “inwardly rectifying” and “outwardly rectifying” categories) (Table 7.1). We will briefly summarize the known characteristics of each type of channel, and then focus in more detail on the inwardly rectifying (Kir) channels which have been shown to be altered in epilepsy and other disease states.
Voltage-Independent K+ Channels
TREK1, TREK2, and TWIK1 are voltage-independent two-pore domain (K2P) K+ channels found in cultured astrocytes and astrocytes in hippocampal slices [7,8]. TREK channels have been found to contribute to the “passive” current pattern in hippocampal astrocytes [7]. TREK channels have various properties including activation by physical (membrane stretch, acidosis, temperature) and chemical (lipids, volatile anesthetics) stimuli. The functional significance of changes in TREK channels in disease states and TREK channels as pharmacologic targets is beginning to be explored in detail in certain pathologies [9–11]. Interestingly, TREK-1 channels can mediate glutamate release on GPCR activation [12] which could affect excitability.
Inwardly Rectifying (Kir) K+ Channels
Kir channels are inwardly rectifying potassium channels expressed in many cell types throughout the body. To date, 15 Kir subunit genes have been identified and classified into seven subfamilies (Kir1.x to Kir7.x). These subfamilies can be categorized into four functional groups: classical Kir channels (Kir2.x); G protein-gated Kir channels (Kir3.x); ATP-sensitive K+ channels (Kir6.x); and K+-transport channels (Kir1.x, Kir4.x, Kir5.x, and Kir7.x) (Figs. 7.1 and 7.2) [13].

(A) Primary structure of the Kir channel subunit (left). Each Kir subunit contains two transmembrane (TM1 and TM2) regions, a pore-forming (H5) loop, and cytosolic NH2 and COOH termini. As a comparison, the structure of voltage-gated K+ (Kv) channel subunit, which possesses six transmembrane (TM1-TM6) regions, is shown on the right. (B) Amino acid sequence alignment and phylogenetic analysis of the 15 known subunits of human Kir channels. These subunits can be classified into four functional groups. Source: Reproduced with permission from H. Hibino, A. Inanobe, K. Furutani, S. Murakami, I. Findlay, and Y. Kurachi. 2010. Inwardly rectifying potassium channels: their structure, function, and physiological roles. Physiol Rev 90:291–366 (Figure 2).

(A) Schematic representation of the structure of a generic Kir channel. The Kir channel is divided into transmembrane and cytoplasmic domains. The NH2 and COOH termini are cytosolic and together contribute to the formation of the cytoplasmic domain. (B) Tetrameric assembly of Kir channels. The molecular architecture of a tetrameric Kir channel (protein database ID 2QKS: Kir3.1-KirBac3.1 chimera) is represented as a cartoon model. The front subunit has been omitted for clarity. The organization of the tetramer of NH2 and COOH termini leads to an extended pore for ion permeation. (C) Transmembrane domain. An enlarged view of the transmembrane domain indicates several important secondary structures for Kir channel function. The transmembrane domain comprises three helices: TM1, pore, and TM2. At the membrane-cytoplasm interface, there is also an amphiphilic slide helix. The residue that is largely responsible for the interaction with polyamines and Mg2+ and thus inward rectification is indicated by the yellow/red spheres (D131 in Kir3.1 which was mutated in the original coordinate). (D) Cytoplasmic domain. The opening of Kir channels requires PtdIns(4,5)P2. Those amino acid residues (blue) associated with the interaction with PtdIns(4,5)P2 are distributed on the surface of the cytoplasmic domain toward the plasma membrane. The center of the cytoplasmic domain is a water-filled cavity that contributes to the ion permeation pathway. Some residues associated with inward rectification (red) map along this cavity. Kir3.x channels are activated by direct interaction with a G protein βγ (Gβγ) subunit and channels containing Kir3.2 and Kir3.4 subunits can also be activated by Na+. The amino acids responsible for these stimuli are indicated in green (Gβγ) and pink (Na+). (C) and (D) have been derived from (B). Source: Reproduced with permission from H. Hibino, A. Inanobe, K. Furutani, S. Murakami, I. Findlay, and Y. Kurachi. 2010. Inwardly rectifying potassium channels: their structure, function, and physiological roles. Physiol Rev 90:291–366 (Fig. 4).
Their inward rectification refers to the voltage-dependence of channel activation: Kir channels are closed when the membrane is depolarized and open at hyperpolarized potentials. This property is due to their sensitivity to Mg2+ and polyamines, which block the channel at depolarized potentials [14]. Increases in extracellular K+ result in inward flux of K+ ions through Kir channels, critical for K+ removal from the extracellular space (ECS). Kir channels are inhibited by micromolar concentrations of Ba2+ and “barium-sensitive” potassium flux is therefore thought to be Kir-mediated (Fig. 7.3).

(A1) Membrane currents were elicited in the whole-cell mode by depolarization and hyperpolarization between –160 and +20 mV (1; 10 mV increments; holding potential, −70 mV; dashed line, zero current). After adding 100 µM Ba2+ to the bath, currents were reduced (2). (A2) Ba2+-sensitive currents were determined by subtracting respective current families (1–2). Ba2+-sensitive currents (inset) reversed at −88.5 mV. (B) The averaged I/V relationship of Ba2+ (100 µM)-sensitive currents obtained in the presence of Cbx of six astrocytes (mean±SD). In each cell, data were normalized to maximum inward currents. Source: Reproduced with permission from G. Seifert, K. Hüttmann, D.K. Binder, C. Hartmann, A. Wyczynski, C. Neusch, and C. Steinhäuser. 2009. Analysis of astroglial K+ channel expression in the developing hippocampus reveals a predominant role of the Kir4.1 subunit. J Neurosci 29:7474–88.
In astrocytes, Kir channels are abundantly expressed and are thought to be largely responsible for setting the resting membrane potential [15,16]. Thus, a primary mechanism for K+ reuptake from the ECS is thought to be via Kir channels. Glial Kir contributes to K+ reuptake and spatial K+ buffering [4,17], which has been most clearly demonstrated in the retina [18–21]. In CNS astrocytes, the expression of Kir4.1 and Kir5.1 has been investigated most thoroughly and have the predominant functional role [22–24] (Fig. 7.4). Kir4.1 is the principal Kir channel expressed in astrocytes, appearing during embryonic development and gradually increasing between the early postnatal period and adulthood [7,25] (Figs. 7.5 and 7.6). Pharmacological or genetic inactivation of Kir4.1 leads to impairment of extracellular K+ regulation [26–28]. In addition, members of the strongly rectifying Kir2 family may also contribute to astroglial K+ buffering [15,29].

(A) Brain astrocytes. Astrocytes harbor and localize homomeric Kir4.1 channels and heteromeric Kir4.1/5.1 channels in particular parts of their membrane: both the homomer and the heteromer are present in perisynaptic processes, and the heteromer is situated alone in the endfeet. (B) Retinal Müller cells. These cells express the heteromeric Kir4.1/5.1 channel at perisynaptic sites and the homomeric Kir4.1 channel at perivascular processes and in endfeet. In both the astrocytes and Müller cells, the Kir channels coexist with the astroglial water channel aquaporin-4 (AQP4) in the same membrane domains (A and B). Source: Reproduced with permission from H. Hibino, A. Inanobe, K. Furutani, S. Murakami, I. Findlay, and Y. Kurachi. 2010. Inwardly rectifying potassium channels: their structure, function, and physiological roles. Physiol Rev 90:291–366 (Fig. 16).

(A1) Kir4.1 immunoreactivity was lacking in Kir4.1−/− mice. (A2) Perikarya of cells were visualized by Nissl staining (blue). (B1, C1, D1) Kir4.1 immunoreactivity (gray levels) in hippocampal slices from mice at postnatal stages as indicated. (B2, C2, D2) Double labeling of Kir4.1 (green) and Nissl (blue). Scale bar, 300 µm. Source: Reproduced with permission from G. Seifert, K. Hüttmann, D.K. Binder, C. Hartmann, A. Wyczynski, C. Neusch, and C. Steinhäuser. 2009. Analysis of astroglial K+ channel expression in the developing hippocampus reveals a predominant role of the Kir4.1 subunit. J Neurosci 29:7474–88.
Outwardly Rectifying K+ Channels
Outwardly rectifying K+ channels (Kv channels) comprise over 40 subtypes [30]. These channels are closed at resting membrane potential but open in response to membrane depolarization. They are not blocked by micromolar Ba2+ but are sensitive to the voltage-dependent K+ channel blockers tetraethylammonium (TEA) and 4-aminopyridine (4-AP). Members of the Kv superfamily include Kv1.4 channels which mediate rapidly inactivating A-type potassium currents (KA) and are responsible for fast hyperpolarization; and Ca2+-dependent K+ channels (KCa), which have a dual gating mechanism by both membrane voltage and cytosolic Ca2+, and have been divided into three types (BK, IK, SK) differing in biophysical characteristics [31,32].
Astrocytes and K+ Homeostasis
A critical role for astrocytes in maintaining extracellular K+ homeostasis was first proposed by Leif Hertz in 1965 [33]. Neuronal activity is accompanied by influx of Na+ and Ca2+ into neurons and efflux of K+ from neurons into the ECS. Due to the limited ECS volume [34], small amounts of K+ released by neurons can significantly affect extracellular potassium concentration. Prolonged increases in extracellular [K+] could prolong neuronal depolarization and inhibit repolarization and therefore undermine neuronal and synaptic function [35].
Therefore, during normal activity extracellular [K+] is tightly regulated by both local K+ uptake and K+ spatial buffering. Local K+ uptake is accomplished by individual astrocytes through ion channels, exchangers, and transporters, notably Kir4.1 but also importantly via Na+/K+/ATPase (sodium-potassium pump) and Na+/K+/2Cl− cotransporters [36,37]. Potassium spatial buffering [4,38,39] involves redistribution of K+ ions throughout the gap-junction-connected glial “syncytium” from areas of high to low concentration, thereby helping to restore basal extracellular K+ levels (Fig. 7.7). According to this model, the difference between glial syncytium membrane potential and local K+ equilibrium potential at sites of high extracellular K+ accumulation drives potassium ions into the astrocytic network. The inwardly rectifying K+ channel Kir4.1 is the primary K+ channel responsible for astrocyte K+ uptake [15,27,40]. Once taken up into astrocytes, K+ can then propagate through the glial network to sites of lower [K+]o [41]. Given the colocalization of Kir4.1 channels and aquaporin-4 (AQP4) water channels, a similar process likely occurs for what might be called spatial “water” buffering, that is water distribution from sites of higher to lower to higher osmotic pressure within the astrocyte network (Fig. 7.8). These processes link water and K+ reuptake into astrocytes leading to astrocyte swelling with intense neuronal activity (see also Chapter 8: Water Channels).

Potassium is released into the extracellular space during neuronal activity (K+ efflux underlies the recovery phase—repolarization—of the action potential). Buffering of extracellular potassium occurs through astroglial inward rectifier potassium channels Kir4.1, Na+/K+/ATPase and Na+/K+/2Cl− cotransporters, which all provide for local potassium buffering. Astrocytes take up excess K+, redistribute the K+ through the astroglial syncytium via gap junctions (spatial potassium buffering), and release K+ through Kir4.1 distantly. Source: Reproduced with permission from Verkhratsky and Butt, Glial physiology and pathophysiology. Wiley-Blackwell, 2013 (Fig. 4.43).

Synaptic activity causes a local elevation in the concentrations of extracellular glutamate, K+, and CO2. Glutamate and K+ are accumulated by astrocytes via glutamate transporters and Kir4.1 channels, respectively, whereas CO2 enters the cell in the form of HCO3− via Na+/HCO3− cotransporter. These events increase osmotic pressure within the astrocyte, which favors water influx through aquaporins, resulting in local swelling of the astrocyte processes and shrinkage of the extracellular space. Locally accumulated water is redistributed through the astroglial network and can be extruded distantly, for example at blood vessels (also through aquaporins), resulting in local shrinkage of the astrocyte processes, and therefore increasing the extracellular volume near the site of efflux. Source: Reproduced with permission from Verkhratsky and Butt, Glial physiology and pathophysiology. Wiley-Blackwell, 2013 (Fig. 4.46).
Genetic knockout mice have provided support for the roles of both astrocyte gap junctions and Kir4.1 channels in regulating extracellular K+ homeostasis. Mice completely deficient in astrocytic gap junction coupling (Cx30/43 double knockout mice) exhibited impaired K+ buffering, spontaneous epileptiform activity, and a decreased seizure threshold [42]. Kir4.1 global knockout mice demonstrated the importance of Kir4.1 in K+ buffering by several cell types, including Müller glia [26], cochlear epithelium [43], and brainstem [28]. Similar results were obtained with RNAi knockdown of Kir4.1 in cultured astrocytes [44]. Generation of a glial-conditional knockout mouse (cKO) of Kir4.1 via Cre-lox technology was performed using the human GFAP astrocyte promoter (gfa2). This led to loss of Kir4.1 throughout the CNS, depolarization of all glial types, impairment of astrocyte K+ and glutamate uptake, ataxia, seizures, and early postnatal lethality [40]. A follow-up in vivo study in the same cKO mice demonstrated the role of Kir4.1 in setting the membrane potential of glial cells and its contribution to astrocyte potassium permeability [45]. In another mouse model with glia-specific Kir4.1 deletion, K+ clearance was delayed after synaptic activation in stratum radiatum of hippocampal slices [46]. Alteration in Kir4.1-mediated K+ uptake may directly impact neuronal plasticity [47]. These studies have clearly indicated the critical importance of astrocytic Kir4.1 in the maintenance of astrocyte membrane potential and overall CNS potassium homeostasis, and the potential epileptogenic effect of K+ dysregulation.
Alterations in K+ Homeostasis in Human Epilepsy
Therefore, in the context of epilepsy it is important to determine whether there are changes in astrocyte K+ regulation. During the intense neuronal activity of seizures, extracellular [K+] may increase from ~3 mM to a ceiling of 10–12 mM [37,48–50]. (Under nonphysiological conditions such as hypoxia/ischemia and spreading depression, extracellular [K+] may rise as high as 40–60 mM [49,51,52].) Any impairment of glial K+ uptake would be expected to be proconvulsant based on many previous studies. In the hippocampus, millimolar, and even submillimolar increases in extracellular K+ concentration powerfully enhance epileptiform activity [53–56]. High-K+ also reliably induces epileptiform activity in hippocampal slices from human patients with intractable temporal lobe epilepsy and hippocampal sclerosis [57]. Since both extracellular K+ concentration and osmolarity have been shown to dramatically modulate neural excitability [58], it is plausible that changes in astrocytic K+ or water channels could contribute to hyperexcitability in epilepsy.
Alterations in Kir Currents and Channels in Epilepsy
Downregulation of astrocytic Kir channels has indeed been found in the injured or diseased CNS. Kir currents are reduced following injury-induced reactive gliosis in vitro [59], entorhinal cortex lesion [60], freeze lesion-induced cortical dysplasia [61,62], and traumatic [63] and ischemic [64] brain injury. In addition, human tissue studies have reported a significant loss of perivascular Kir4.1 expression (~50%) and function in TLE patients, suggesting impaired spatial K+ buffering in the epileptic hippocampus [65–71]. Reduction in Kir conductance in astrocytes [69] and potassium clearance [71] were observed in human epilepsy specimens. Interestingly, two studies directly examined astrocytic K+ currents in sclerotic versus nonsclerotic hippocampi resected from epileptic patients. Using ion-sensitive microelectrodes, Heinemann’s group compared glial Ba2+-sensitive K+ uptake in the CA1 region of hippocampal slices obtained from patients with or without mesial temporal sclerosis [68,72]. Ba2+, a blocker of Kir channels, augmented stimulus-evoked K+ elevation in nonsclerotic but not in sclerotic specimens, suggesting an impairment in Kir-mediated K+ buffering in sclerotic tissue. Direct evidence for downregulation of Kir currents in the sclerotic CA1 region of hippocampus came from Steinhäuser’s group who found, using comparative patch-clamp analysis, that there was a reduction in astroglial Kir currents in sclerotic compared to nonsclerotic hippocampi [67]. These data indicate that dysfunction of astroglial Kir channels could underlie impaired K+ buffering and contribute to hyperexcitability in epileptic tissue [73]. More recently, Heuser et al. found that the loss of astrocytic Kir4.1 expression was most pronounced around vessels in gliotic areas of the sclerotic hippocampus of mesial temporal lobe epilepsy (MTLE) patients [66]. Thus, increasing evidence suggests that the dysregulation of K+ spatial buffering may play a role in hyperexcitability. Direct evidence for a potential causative role of reduced Kir4.1 channel expression in epileptogenesis derives from an albumin model of BBB disruption in which Kir4.1 downregulation occurs prior to onset of epilepsy; [74,75] however, other studies report no changes in astrocytic Kir currents 7–16 days following systemic kainic acid injection [76] and increased Kir4.1 expression in a rat pilocarpine model [77]. Kir4.1 may also be regulated by inflammatory cytokines such as interleukin (IL)-1β [78] which are known to play a role in epilepsy (see Chapter 13: Inflammation).
Further evidence for a role of alteration in Kir4.1 function in human epilepsy arises from genetic association studies. Quantitative trait loci mapping in mice led to identification of genetic variation in the Kir4.1 gene Kcnj10; this led to human genetic association studies which identified an association between the human Kir4.1 gene KCNJ10 mutations and seizure susceptibility in human populations [79,80]. Further evidence was adduced for an allelic association with variations in KCNJ10 and idiopathic generalized epilepsy [81,82]. Interestingly, human subjects were subsequently found with homozygous loss-of-function mutations in KCNJ10 leading to the definition of a specific autosomal recessive syndrome consisting of Epilepsy, Ataxia, Sensorineural deafness, and Tubulopathy (EAST syndrome) [83,84], a different version of which was also termed SeSAME syndrome (Seizures, Sensorineural deafness, Ataxia, Mental retardation, and Electrolyte imbalance) [85], both together termed SeSAME/EAST syndrome [86]. The combined phenotype of this syndrome parallels well the expression of Kir4.1 in the brain, inner ear, and kidney. A subset of patients with autism and epilepsy were found to have gain-of-function mutations of KCNJ10 [87]. Single nucleotide variations in the KCNJ10 gene have also been found in TLE patients with hippocampal sclerosis [88].
Diverse K+ Channelopathies in Animal and Human Epilepsies
It is important to recognize that aside from Kir4.1/KCNJ10, a wide variety of mutations in K+ channels have been described in distinct forms of animal and human epilepsies [89] (Tables 7.2 and 7.3). However, as most K+ channels are not specific to astrocytes and are also expressed by neurons, a detailed discussion of each of these K+ channel mutations and syndromes is beyond the scope of this chapter and is not pathophysiologically limited to astrocytes. Kir4.1/KCNJ10 mutations represent a “purer” astrocytic contribution to epilepsy. Nevertheless, it is instructive to note the powerful impact of K+ channel mutations—both neuronal and astrocytic—have on excitability and seizure susceptibility. In the case of Kir4.1 loss-of-function mutations, seizure susceptibility likely arises from abrogation of the K+ buffering mechanisms described above; whereas in the case of Kv channel mutations in neurons, seizure susceptibility is likely more related to loss of neuronal repolarization [90]. Interestingly, one of the newest and most promising antiepileptic drug mechanisms of action is that of retigabine, which activates voltage-dependent K+ channels of the Kv7 subfamily and is thus a “neuronal potassium channel opener” [91]. No similar drug yet exists to activate glial Kir channels.
Table 7.2
K+ Channelopathies in Human Epilepsies
Channel | Gene/Protein | Expression in Brain Regions Relevant to Epilepsy | Epilepsy Type | Gene Mutation/Channel Dysfunction | References |
Voltage-gated K+ channels | KCNA1/Kv1.1 (pore forming α subunit) | Axons/terminals of hippocampal neurons (Shaffer collateral axons and mossy fibers contacting CA3 neurons); hippocampal interneurons of hilus and CA1; neocortical pyramidal neurons | Generalized and partial seizures associated to EA1 | Loss-of-function mutations generally associated with reduced current amplitudes; positive shift of the activation V1/2; increased sensitivity to Zn2+ inhibition | Assaf and Chung (1984); Browne et al. (1994); Adelman et al. (1995); D’Adamo et al. (1998, 1999); Zuberi et al. (1999); Geiger and Jonas (2000); Cusimano et al. (2004); Imbrici et al. (2006, 2007, 2008); Guan et al. (2006) |
KCNAB2/Kvβ2 (β accessory subunit for Kv1 channels) | Widely expressed in cerebral cortex and hippocampus | Severe epilepsy including infantil spasms | Allele diletion/haploinsufficiency | Heilstedt et al. (2001) | |
LGI1 (accessory protein for Kv1 channels) | Neocortex and hippocampus | Autosomal dominant lateral temporal lobe epilepsy | E383A, frameshift with protein truncation/Mutated LGI1 does not prevent Kvβ1-mediated Kv1 channel inactivation, a function performed by the WT protein | Kalachikov et al. (2002); Morante-Redolat et al. (2002) | |
KCND2/Kv4.2 (IA pore forming α subunit) | Dentrites of hippocampal neurons | Temporal lobe epilepsy | Truncated Kv4.2 subunit/attenuated IA current density | Singh et al. (2006) | |
KCNV2/Kv8.2 (silent subunit associating with Kv2 channels) | Pyramidal neurons and principal excitatory neurons of the pyramidal cell layers and the dentate gyrus; cortex, with high levels of transcript in layers 2/3 and 5 | Febrile and afebrile partial seizures; epileptic encephalopathy | R7K, M285K/Reduction of Kv2.1 mediated current; M285K impairs the voltage-dependence of the channel | Jorge et al. (2011) | |
KCNQ2-3/Kv7.2-3 (M current pore forming α subunit) | Widely expressed in brain at neuronal cell bodies | Benign familial neonatal convulsions | Five-base pair insertion deleting more than 300 amino acids from the KCNQ2; missense mutations in critical regions for KCNQ3 channel function / Reduced KCNQ current amplitudes | Biervert et al. (1998); Charlier et al. (1998); Schroder et al. (1998); Singh et al. (1998) | |
KCNH2/Kv11.2 (HERG channel pore forming α subunit) | Widely expressed in brain | Epilepsy associated with type 2 long QT syndrome | Loss-of-function mutations | Keller et al. (2009); Omichi et al. (2010); Tu et al. (2011); Zamorano-León et al. (2012) | |
Ca2+-dependent K+ channels | KCNMA1/KCa1.1 (BK channel pore forming α subunit) | Axons and presynaptic terminals of excitatory neurons of cortex and hippocampus | Generalized epilepsy and paroxysmal diskynesia | D434G/Increase of channel open probability and calcium dependence of KCa1.1 expressed alone or with KCaβ1, KCaβ2, or KCaβ4; loss-of-function of KCa1.1/KCaβ3-mediated currents | Du et al. (2005); Díez-Sampedro et al. (2006); Lee and Cui (2009); Yang et al. (2010) |
KCNMB3/KCaβ3 (accessory protein for KCa1.1 channels) | Widely expressed at low levels in brain | Idiopathic generalized epilepsy | Loss-of-function of KCa1.1/KCaβ3-mediated currents | Behrens et al. (2000); Hu et al. (2003); Lorenz et al. (2007) | |
Inwardly rectifying K+ channels | KCNJ2/Kir2.1 (pore forming α subunit) | Hippocampus, caudate, putamen, nucleus accumbens; to lower levels in habenula and amygdale | Seizures associated to the Andersen Tawil Syndrome (ATS) | Loss-of-function mutations with dominant-negative effects | Haruna et al. (2007); Chan et al. (2010) |
KCNJ10/Kir4.1 (pore forming α subunit) | Oligodendrocytes and astrocytes surrounding synapses and blood vessels, mainly in the cortex, thalamus, hippocampus, and brainstem | Seizure susceptibility | R271C missense variation; no alteration in the biophysical properties of the channel when heterologously expressed | Buono et al. (2004); Connors et al. (2004); Shang et al. (2005) | |
Epilepsy associated to EAST syndrome | Loss-of-function recessive mutations | Bockenhauer et al. (2009); Scholl et al. (2009) | |||
Epilepsy associated to autism spectrum disorders (ASDs) | R18Q, V84M, gain-of-function of Kir4.1 and Kir4.1/Kir5.1-mediated current | Sicca et al. (2011) | |||
KCNJ11/Kir6.2 (KATP channel pore forming α subunit) | Hippocampus (principal neurons, interneurons, and glial cells); neocortex, entorhinal, and piriform cortex | Developmental delay, epilepsy, and neonatal diabetes mellitus (DEND syndrome) | Gain-of-function mutations leading to decreased channel inhibition by ATP, or enhanced Mg2+-nucleotide-induced activation | Karschin et al. (1997); Hattersley and Ashcroft (2005) | |
ABCC8/SUR1 (KATP channel accessory regulatory subunit) |
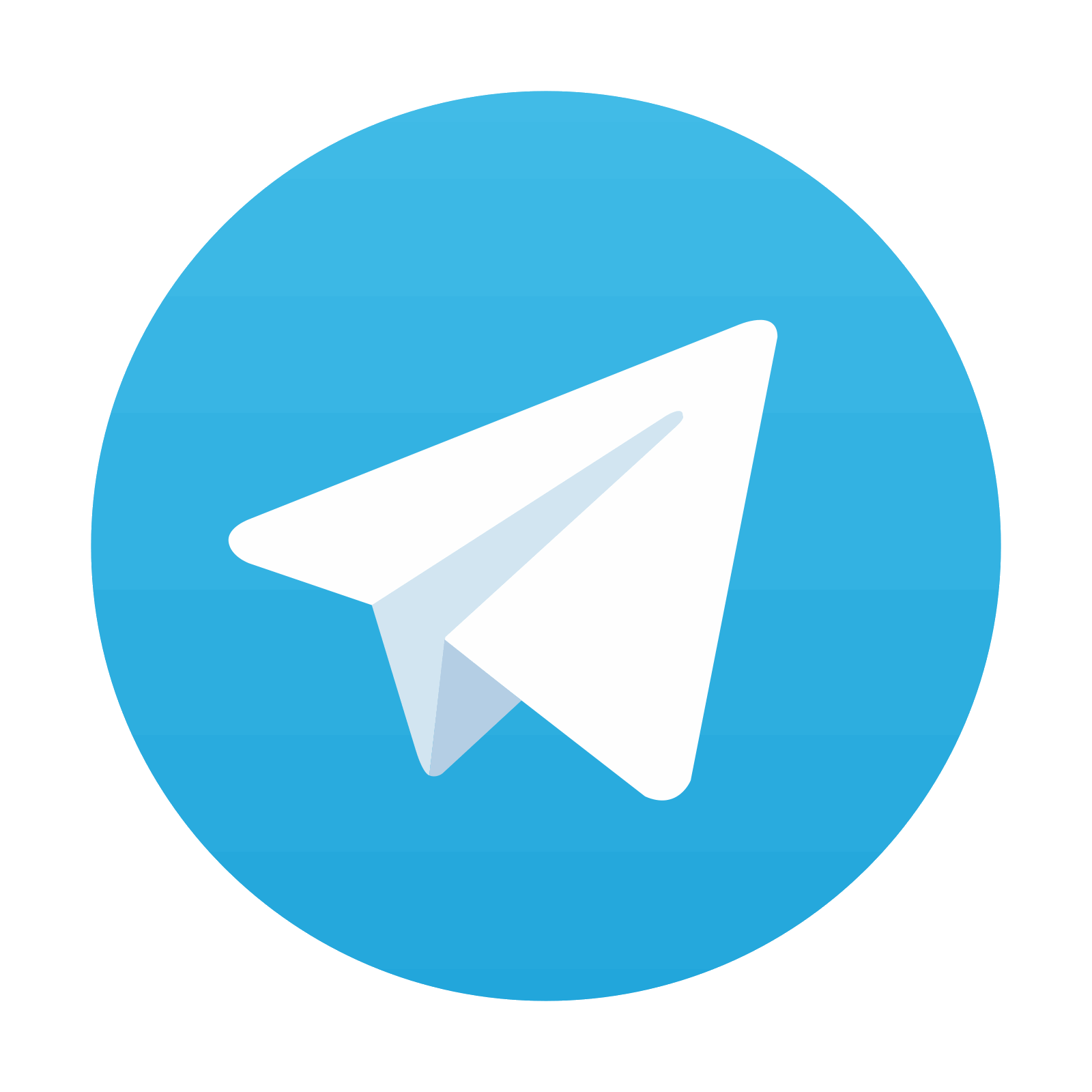
Stay updated, free articles. Join our Telegram channel
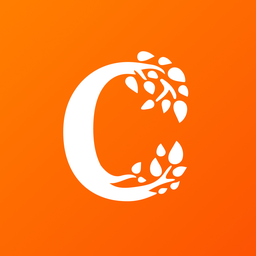
Full access? Get Clinical Tree
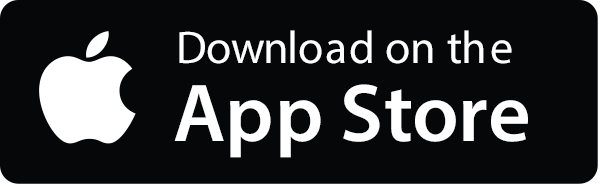
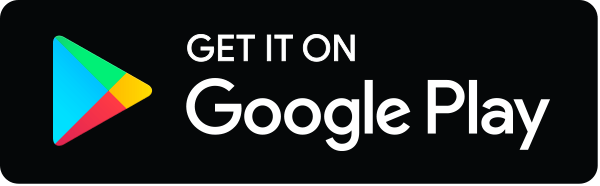
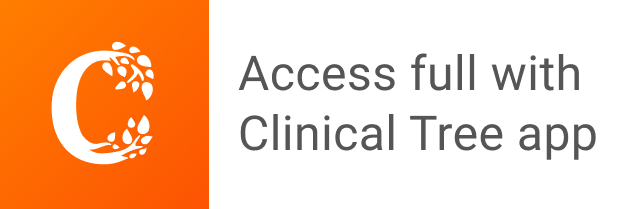