Promising Developments in Stroke Imaging
Pearls
Stroke triage requires rapid yet comprehensive neurovascular imaging.
Computed tomography (CT) protocols should provide time-resolved CT angiography of the head and neck and CT perfusion of the brain to facilitate diagnosis and treatment selection.
Magnetic resonance (MR) approaches now produce noninvasive, multidimensional, time-resolved vascular imaging data to assess anatomy, flow patterns, velocities, and pressure gradients.
Angiography systems now offer a unified technical platform to image brain parenchyma and vessels, thereby facilitating combined diagnosis and intervention.
♦ Overview of Future Imaging
The future of stroke imaging is bright, multifaceted, and central to the diagnosis and management of patients with neurovascular disease. This chapter outlines some of the logical extensions of current computed tomography (CT), magnetic resonance (MR), and angiographic techniques. We cover both the state of the art in current practice as well as our “blue-sky” vision of future imaging developments related to ischemia and hemorrhage in the brain. Undoubtedly, other technologies, including targeted molecular imaging, will be incorporated as these methods mature. As with any scientific development, advancement in the field requires not only technical improvements but also a mindset and willingness to evaluate and embrace new work flow, diagnostic approaches, and treatment algorithms. Growth in this area is a synergistic parallel development process because new imaging methods promote and enable new treatment possibilities, and vice versa. Imaging advances are crucial to improved patient outcomes both today and tomorrow.
Neurovascular Computed Tomography
From the perspective of acute stroke triage based on CT, the future is here already. A 10-minute protocol can already screen brain parenchyma, blood vessels of the head and neck, and brain perfusion. In some ways, advanced technical abilities of current and emerging modalities already produce more information than we know what to do with in practice. Such a rich data set provides a solid basis for patient triage and management of either ischemic or hemorrhagic stroke. The next major advances in this area will include new scanner hardware such as flat panel machines with extended z-axis coverage. Here we will see a technical convergence with angiographic platforms. New CT scanners will be deployed with multi-energy, undersampled data acquisition protocols and advanced noise-reduction reconstruction algorithms to produce low-dose, multidimensional data sets. Such an approach will yield time-resolved computed tomography angiography (CTA) and CT perfusion data from the heart to the top of the head in a 1-minute examination. Fully automated postprocessing will be done at the scanner console for immediate review.
Clinical implementation of any new advanced technology must consider not only the diagnostic performance of the technique itself but how and when to deploy it. This is particularly true with respect to the timing of acute stroke imaging; it is not just the question of what test to do, but of when to do it. It can be argued that comprehensive CT and magnetic resonance imaging (MRI) neurovascular protocols should be done immediately on first presentation with suspected neurovascular disease. Patients with either acute ischemic or hemorrhagic conditions in the brain benefit from immediate, rapidly performed, and fully comprehensive stroke protocols to address the brain parenchymal patterns of injury, relevant vascular lesions of both the head and neck, and perfusion characteristics. Such comprehensive imaging data can be useful in acute triage and in the selection of therapy based on the individual patient’s physiology and anatomy rather than on the clinical features or arbitrary time windows.1 In stroke or transient ischemic attack (TIA), it has been shown that rapid referral for treatment of underlying vascular lesions also leads to improved outcome with reduction in secondary strokes. If an examination is ultimately appropriate for a patient with acute stroke, why not perform it immediately from the emergency room, rather than a few days later, and have the information available for definitive management from that point forward?

A suggested acute stroke triage algorithm using advanced CT and MR protocols is shown in Fig. 12.1 . This incorporates current therapies including intravenous tissue-type plasminogen activator (tPA) as well as approved endovascular devices and other intraarterial interventional strategies. Current and future efforts must streamline the work flow from the moment the patient reaches the emergency room until the definitive treatment is administered. With the realization that up to 2 million neurons die per minute in a typical middle cerebral artery occlusion, every second counts in both data acquisition and postprocessing.2 Quality data must be rapidly produced and conveyed to the treatment team to facilitate fast yet fully informed decisions.
In our hospital, automated software running at the MR scanner console has been quickly providing multiparametric perfusion data for the past 10 years ( Fig. 12.2 ). Postprocessing of color maps requires no physician input or specialized training, takes about 1 minute per scan, and maps are sent to the picture archiving and communication system (PACS) before the patient is rolled out of the MR suite. Such approaches, when combined with diffusion data, form the basis for treatment based on mismatch, or so-called penumbral imaging.3 Treatment decisions are refined by knowledge of the vascular occlusion anatomy and filling patterns provided through fast time-resolved CTA or magnetic resonance angiography (MRA).
Innovations in Magnetic Resonance Angiography Stroke Evaluation
Imaging of the cerebrovascular system is a critical step in the evaluation of patients presenting with symptoms of stroke. A clinically desirable MRA stroke protocol would include features such as short examination time, four-dimensional (4D) temporal resolution, high spatial resolution, volumetric whole brain coverage, no or minimal risk to the patient, robustness, insensitivity to patient motion, and the ability to assess hemodynamic changes in vivo. In this section we present techniques in development that will improve the performance of MRA in stroke patients. In addition, we discuss HYPRFlow angiography as a comprehensive MRA method to evaluate stroke patients.
The MR systems of the future must be able to match the speed, ease of access, and reliability of CT if MR is to continue to play a major role in the evaluation of patients with stroke. MR systems will be increasing sited in the emergency department and staffed 24 hours a day, 7 days a week. The examinations will be transmitted to members of the stroke team simultaneously and contemporaneously with the imaging. The images will be viewed on hand-held devices that resemble small netbooks and will activate when an acute stroke patient has been identified. The hand-held devices will also permit voice and text communication among the members of the stroke team and instantaneous access to online tools.
Barriers to using MR in the emergency department (ED) are eliminated in the future through the use of revolutionary new procedures and technologies developed to quickly screen patients. This technology will be adapted from new methods to screen passengers prior to boarding airline flights. The screening process will be further enhanced by the widespread use of MR-compatible clips, pacemakers, and stimulators. The screening will be completed during patient transport to the hospital and will be confirmed by a physician member of the stroke team. Thus the patients will quickly be made eligible for the MR examination.

Following clinical evaluation, the patient is prepared to be placed into the ultra-wide-bore, 3-tesla magnet. The patient is placed on a detachable ED/MR table that has intravenous pumps, anesthesia equipment, oxygen, electrocardiogram (ECG), and other support systems integrated into the table. The interior of the magnet has been modified to greatly increase the bore size and decrease the length of the bore. This has been accomplished by creating a gradient system that is only a few millimeters in thickness. The gradients enable echo times of less than 1 millisecond and repetition times of less than 2 milliseconds, roughly twice as fast as today’s gradient systems. The dB/dT and SAR concerns are minimized though innovative coil and pulse sequence design. There is no sound produced by the scanner. Remote patient monitoring is easy to achieve via wireless telemetry.
The ED/MR table is constructed with a comfortable and conformable coil system. The global array has 128 channels and covers the heart, aortic arch, neck, and head in one coil system. The coils are divided into a 32-channel cardiopulmonary subgroup, a 32-channel aortic arch subgroup, a 32-channel neck subgroup, and a 32-channel head subgroup. The coil system is designed to provide high-resolution images from the heart to the brain. Cardiac imaging is routinely included to search for sources of emboli, eliminating the need for additional testing such as echocardiography. The multiple arrays generate images with high spatial resolution over a large anatomic region.
After placing the patient into the coil system and connecting the appropriate support systems, the patient is moved into the magnet room for a 15-minute comprehensive stroke evaluation. One might wonder how so much data can be acquired in only 15 minutes. There are several acceleration methods that can accomplish this. These include short repetition times, parallel imaging, radial undersampling, compressed sensing, random k-space sampling, and other methods to acquire the minimum data necessary to provide the image quality needed for diagnosis.
These cutting edge developments have their genesis in observations made decades ago. When Harry Nyquist was an engineer at AT&T, he wrote 12 articles on signal transmission. His publications led to the development of the Nyquist theorem, which states that the highest frequency in a transmission that can be accurately represented is less than one half of the sampling rate. If the sampling rate is too low, aliasing will occur and the signal (audio waves in Nyquist’s day) will be distorted. Thus, for over two decades MR scientists assumed that a MR scan needed to be nearly fully sampled to prevent aliasing and suppress image artifacts. What the MR community did not realize is that meaningful images can be created with very little data and that artifacts can be minimized if the structures of interest within the imaging volume are sparse. The Nyquist theorem provides a sufficient condition, but not a necessary one, for perfect reconstruction. Vascular structures are sparse within the brain, and thus MRA lends itself to acceleration techniques such as undersampled radial imaging and compressed sensing.

The first innovation to dramatically accelerate stroke imaging is related to the 128-channel coil array.4 , 5 Signal from each coil is used to provide additional spatial encoding. Acceleration is achieved by reducing the number of phaseencoding steps (k-space lines) and substituting the spatial information acquired from the coil array. Pruessmann et al6 introduced sensitivity encoding (SENSE), a parallel imaging method in which the data are first Fourier transformed, resulting in aliased images. The images are then unwrapped by using the spatial information from the coil sensitivity profiles. The SENSE calculations are conducted in image space, and a coil-sensitivity calibration (coil map) is required to carry out the calculations. Figure 12.3 demonstrates the excellent spatial resolution than can be obtained using three-dimensional (3D) phase-contrast MRA and parallel imaging.
An alternate approach includes the coil-sensitivity calibration during the scan, and the calculations are performed in k-space. There are some advantages to this approach; it has a slightly better signal-to-noise ratio (SNR) and can cover a larger field of view.7 Using very short repetition times (TRs) and parallel imaging techniques, one can predict at least a four- to eight-fold acceleration in MRA.
When parallel imaging is used, the coil performance must also be considered. The geometry factor (g factor) will impact the noise in the images. Currently coils are constructed out of conventional materials (such as copper wire). Future coils will use superconducting receivers with two to four times the performance of current systems.
Even higher levels of acceleration can be achieved through undersampling. In the brain, the vessels are relatively sparse within the imaging volume. Sparsity is a feature that we can exploit to our advantage. Because the vessels are sparse within the imaging volume, we can generate images of the vessels by acquiring only a small amount of signal from the volume of interest. A strategy developed by Mistretta’s group8 uses radial acquisition and projection reconstruction to acquire signal from vascular structures in a fraction of the imaging time required for Cartesian imaging.
Radial readout trajectories have several advantages over the rectilinear Cartesian approach that has dominated MRI for decades. The most compelling advantage is that the timeconsuming phase-encoding stepping process is eliminated in 3D radial acquisition. For 3D radial imaging the spatial resolution is defined by the field of view and the readout gradient. For example, a 256 readout at a 25.6-cm field of view (FOV) produces isotropic 1 mm3 voxels, and a 512 readout using the same FOV provides isotropic 0.5 × 0.5 × 0.5 mm voxels. In both instances there is no phase encoding. In fact, by adjusting the readout bandwidth, both acquisitions can be obtained in the same imaging time! The elimination of phase encoding removes a fundamental barrier to image acceleration. A logical question is why was radial acquisition not pursued earlier. The answer is that fully sampled radial acquisition is slightly less efficient than fully sampled Cartesian imaging. However, when radial readout trajectories are used, the acquisition can be vastly undersampled with minimal loss of image quality. The huge advantage of radial imaging is that the undersampling artifacts are either propagated outside of the region of interest or the artifacts contribute to a slight increase in noise in the image. Vastly undersampled radial imaging can be used to obtain gated phase-contrast MR examinations of the entire head with 0.7 × 0.7 × 0.7 mm resolution in less than 5 minutes. A similar examination using Cartesian rectilinear readout and phase encoding would take over an hour.
Radial 3D phase-contrast MRA9 is similar to previous implementations in that stationary background tissue is subtracted from the angiographic images, and quantitative measurements of velocity are obtained from the velocity-induced phase shift. The velocity measurements are used to display hemodynamic conditions such as wall shear stress, relative pressure gradients, flow streamlines, and particle paths. Examples of 3D radial phase-contrast MRA examinations are shown in Figs. 12.4 and 12.5 .
Radial undersampling is only one novel angiographic approach. Undersampling acquisition methods that are under investigation are compressed sensing and variable density sampling. Compressed sensing, also known as compressive sensing, compressive sampling, and sparse sampling, is a technique for acquiring and reconstructing a signal utilizing the prior knowledge that it is sparse or compressible. The concept has existed for at least four decades, but recently the field has exploded.

If we think of another compression method, JPEG, a format commonly used for photographic images, the image data are routinely compressed by a factor of 10 with minimal loss of diagnostic information. So the question is how much information is really necessary to create the MRA image. For compressed sensing to work, the structures of interest must be sparse in the imaging or temporal domain. In addition the sampling method should produce incoherent artifacts when undersampled, and a reconstruction is needed to suppress noise-like artifacts and isolate the sparse vessel signal. For brain MRA the vessels are sparse in the imaging domain and can be completely reconstructed from a highly undersampled examination. Acceleration factors of over 50 are possible. When combined with parallel imaging and ultrashort TRs, a high-resolution MRA of the head can be acquired in less than 1 second.10 , 11

Variable-density k-space sampling is a method to reduce aliasing artifacts in MR images. Because most of the energy of an image is concentrated around the k-space center, aliasing artifacts contain mostly low-frequency components if the k-space is uniformly undersampled. On the other hand, because the outer k-space region contains little energy, undersampling that region does not contribute severe aliasing artifacts. Therefore, a variable-density trajectory may sufficiently sample the central k-space region to reduce lowfrequency aliasing artifacts and may undersample the outer k-space region to reduce scan time and to increase resolution. Variable-density sampling methods have been implemented for spiral imaging and 2D and 3D Fourier transform imaging. This method can significantly reduce the total energy of aliasing artifacts. In general, this method can be applied to all types of k-space sampling trajectories.12
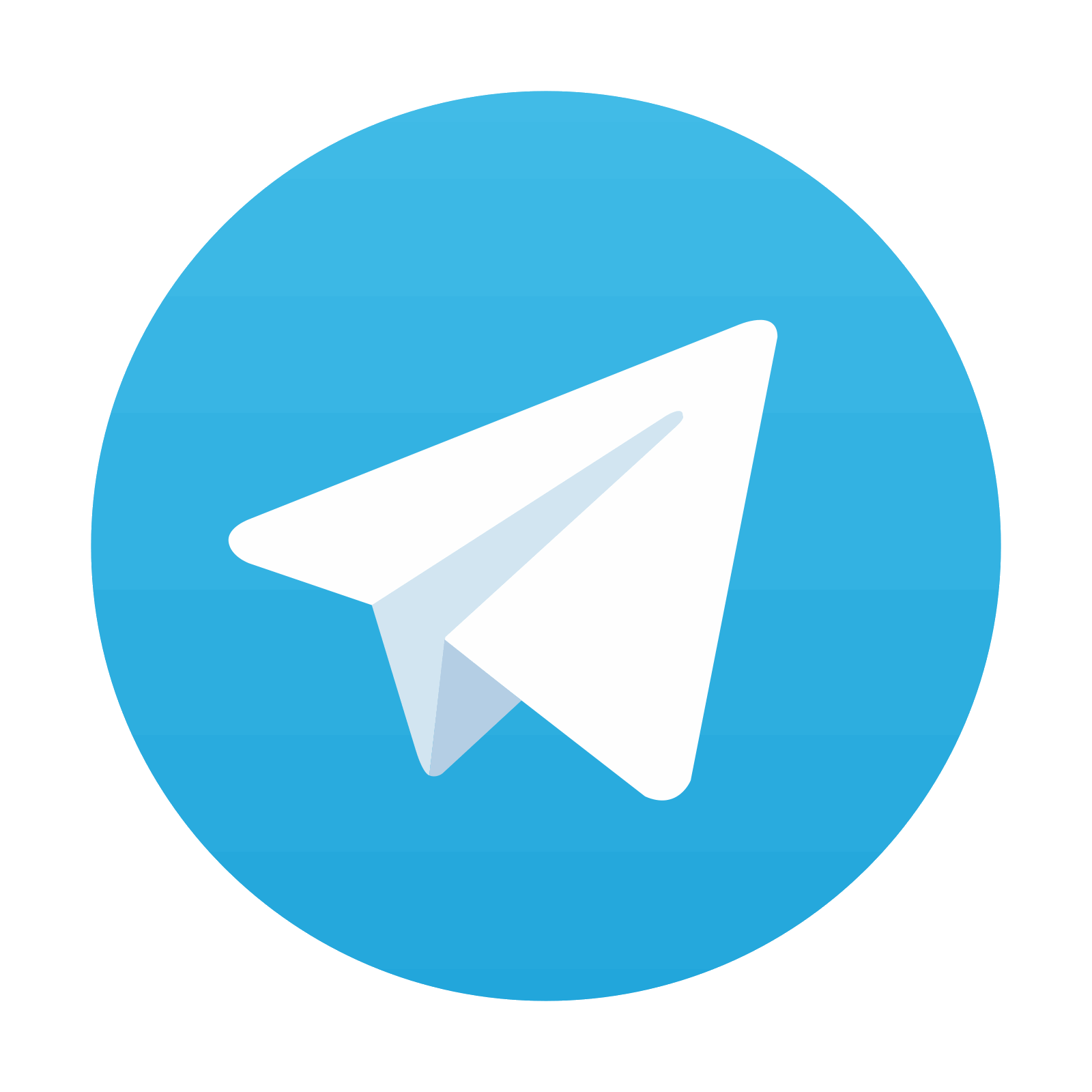
Stay updated, free articles. Join our Telegram channel
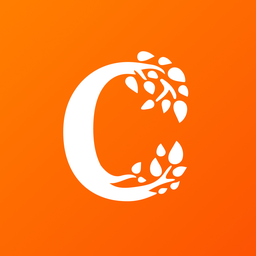
Full access? Get Clinical Tree
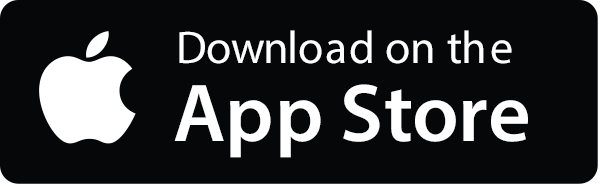
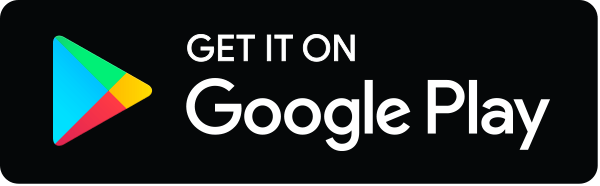
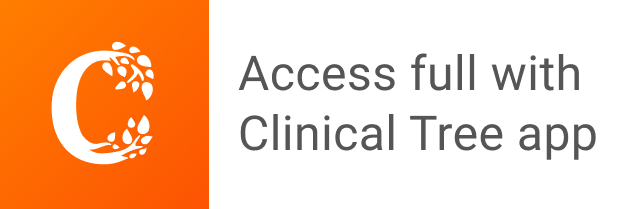