Abstract
The development of a stable intracortical recording electrode has tremendous implications for the rapidly expanding field of neuroprosthetics and, more broadly, brain–machine interfaces (BMIs). A variety of microelectrode designs have been developed during the past two decades, with the ultimate goal to produce a minimally invasive electrode with high spatial resolution. Currently, the majority of BMI tasks rely on intracortical (invasively implanted) microelectrodes that demonstrate poor chronic neural recording performance and reliability, thus limiting BMI from reaching its full potential for use in mainstream clinical applications. While a number of failure modes have been studied, the neuroinflammatory response, in particular, has received significant attention. In this chapter, the motivation, progress, challenges, and prospects for chronically stable intracortical recording electrodes are discussed.
Keywords
Brain–computer interface, Brain–machine interface, Microelectrode, Neuroinflammation, Neuroprosthetic
Outline
Motivation, Progress, and Challenges for Intracortical Recording Electrodes 394
Commonly Used Electrodes for Intracortical Recording 395
The Utah Electrode Array 396
Michigan-Style Microelectrodes 397
Microwires 397
Electrocorticography 397
Electroencephalography 397
Optical Microelectrodes 398
Summary of Intracortical Recording Electrode Failure Mechanisms 398
Neuroinflammatory Underpinnings 398
The Biological Response to Electrode Implantation 398
Overview 398
Soluble Factors Kill Neurons, Degrade and Corrode Implanted Materials, and Maintain the Inflammatory Response 399
Insoluble Factors Serve a Vital Role in Healing and Regeneration but Also Isolate Neurons Electrically and Physically From the Recording Electrode 400
Neuronal Loss at the Electrode–Tissue Interface Attenuates the Source Signal 400
Intracortical Electrode Technologies to Evade the Inflammatory Response and Improve Long-Term Performance 400
Drug Administration 400
Drug/Coating Combinations 402
Material Selection/Surface Properties, Coatings 402
Cell Adhesion Proteins 402
Hydrogel and Other Nonfouling Surfaces 403
Soluble Factor (Cytokine) Sinks 403
Conductive Polymers 403
Material Selection/Bulk Properties 403
Implantation Technique and System Design 404
Avoidance of Vasculature 404
Shape and Speed of Insertion 405
Floating Versus Tethered Lead Wires 405
Perspectives on the Future of Neural Recording Interfaces 405
Acknowledgments [CR]
References 406
Acknowledgments
The authors thank Erika Woodrum and the FES Center for their assistance in preparing illustrations.
This work was supported by the Office of the Assistant Secretary of Defense for Health Affairs through the Peer Reviewed Medical Research Program under Awards W81XWH-15-1-0607 and W81XWH-15-1-0608. Opinions, interpretations, conclusions, and recommendations are those of the authors and are not necessarily endorsed by the Department of Defense. Additionally, this study was supported by Merit Review Award # B1495-R (Capadona) and the Presidential Early Career Award for Scientist and Engineers (PECASE, Capadona) from the United States (US) Department of Veterans Affairs Rehabilitation Research and Development Service. The contents do not represent the views of the U.S. Department of Veterans Affairs or the United States Government. Finally, funding was also received from the National Institute of Health, National Institute of Neurological Disorders and Stroke, (Grant # 1R01NS082404-01A1). None of the funding sources aided in collection, analysis, and interpretation of the data, in writing of the book chapter or in the decision to submit for publication. The authors have no conflict of interest related to this work to disclose.
This chapter outlines and discusses the history and current state of the neural recording field, specifically in the context of cortical neural prostheses. For in-depth reviews of the subject matter, the reader is referred to excellent reviews by , and . Topics covered in this chapter include:
- 1.
Motivation, progress, and challenges for intracortical recording electrodes
- 2.
Commonly used electrodes for intracortical recording
- 3.
Summary of intracortical recording electrode failure mechanisms
- 4.
The biological response to electrode implantation
- 5.
Intracortical electrode technologies to evade the inflammatory response and improve long-term performance
- 6.
Perspectives on the future of cortical neural recording interfaces
Motivation, Progress, and Challenges for Intracortical Recording Electrodes
Motivation
The development of a stable intracortical recording electrode has tremendous implications for the rapidly expanding field of neuroprosthetics and, more broadly, brain–machine interfaces (BMIs). BMIs have significant potential to reduce the burden associated with paralysis and limb loss. By bypassing damaged regions of the nervous system, BMIs offer the promise of reducing the burden of injury and enabling injured individuals to live fuller and more interactive lives ( ). Unfortunately, microelectrodes that are currently used for BMI applications demonstrate poor chronic neural recording performance and reliability. Signal-to-noise ratio degrades over several weeks to months ( ), resulting from a multifactored failure mechanism having both electromechanical (abiotic) and host tissue (biotic) root causes ( ).
In part due to the potential for BMI applications and the need for further development, on April 2, 2013, President Obama launched an initiative to “accelerate the development and application of new technologies that will enable researchers to… show how individual brain cells and complex neural circuits interact at the speed of thought.” In response to this grand challenge, the director of the National Institutes of Health (NIH) released the scientific vision of the Brain Research through Advancing Innovative Neurotechnologies (BRAIN) Working Group, which asked for $4.5 billion over the next 10–12 years to support a strategy for addressing that goal. The BRAIN Initiative looks beyond the current rehabilitative applications proposed for BMIs, to a comprehensive, mechanistic understanding of cognition, emotion, perception, and action in health and disease. The first 5 years of the NIH vision focus largely on developing new “platform” tools and technologies to advance human neuroscience and integrate them into therapeutic interventions. With notable supporters like National Science Foundation (NSF), US Food and Drug Administration (FDA), Defense Advanced Research Projects Agency (DARPA), and many private organizations, the magnitude of the potential impact of the NIH BRAIN Initiative has been compared with that of the Apollo Program and the Human Genome Project ( ).
Progress
Intracortical recording electrodes were initially developed in the 1940s to advance our basic understanding of the nervous system ( ). The first devices consisted of glass pipette and microwire electrodes and were used to interrogate the cortical electrophysiology of various animal models. Since that time, the field has achieved significant strides, both in fabrication technologies and in advancing medical applications of intracortical recording electrodes.
For the past two decades, hundreds of publications have been added to the literature involving “intracortical recording” (or “intracortical microelectrodes”), with over one-third being published since 2010 ( Fig. 28.1 ). With the initiation of more than 120 funded projects stemming from the BRAIN Initiative, this number is set to soar exponentially in the coming years ( ). Today, there are intracortical microelectrodes that have FDA approval (namely the Utah Array from Blackrock). Additionally, there are a broad range of advanced materials and devices with novel capabilities in the preclinical pipeline for future implementation and approval. Recent clinical trials have demonstrated tremendous success, enabling quadriplegic patients to feed themselves, not only with robotic arms ( ) but also with their own muscles with applied stimulation ( ). Very recent evidence even points to the possibility that brain–computer interfaces may be able to trigger partial lower limb recovery in paraplegic patients ( ). Wireless communication has been integrated into new generations of devices that simplifies their clinical implementation and overcomes tethering challenges ( ). The use of optogenetic techniques to allow neural recording or stimulation with light currently remains in preclinical study, but may someday soon provide a means for less invasively or more selectively interacting with neural circuits ( ). The pace of innovation and the achievement of milestones is astonishing.

While the field has made significant progress, BCI has yet to break into the mainstream. To achieve a reliable and natural brain control paradigm, neural signals must be recorded with high-quality and fine spatial resolution such that neuron populations can be triangulated, decoded, and correlated with a person’s intended movement ( ). Such applications often rely on intracortically implanted microelectrodes. Due to the moderate risk associated with implantation surgery and the limited longevity of current recording configurations, one must consider and balance risk to the patient (or animal) with the potential benefit it may serve. The Holy Grail of neural recording interfaces has yet to be perfected: a device that is minimally invasive but can still provide high-density, chronically stable signals.
Challenges
A central focus of neuromodulation has been on the development of electrodes for stimulation applications . Surface chemistries and stimulation paradigms are optimized to balance charge delivery and prevent dissolution and corrosion of the electrode contacts. The host tissue response (including inflammation and encapsulation) is an important consideration, but resulting changes can often be compensated for by tuning stimulation settings (e.g., amplitude, pulse width, direction, etc.) to achieve the desired functional outcome: excitation or blockade of surrounding neurons.
For intracortical recording applications, s ome unique considerations regarding the neural electrode must be made. Researchers working in the field are challenged with a dynamically changing environment, which includes biological, material, and electrical factors, that can be roughly grouped into biotic and abiotic in origin. For a more complete discussion of failure mechanisms, the reader is referred to , and .
Biotic . In recording applications involving implanted neural electrodes, the host tissue response plays a much larger role in determining long-term recording performance and stability than it does in stimulation applications. Inflammation, the healing response, and encapsulation result in changes to the physical and electrical properties of the tissue ( ). Cellular composition and the normal pattern of neural activity surrounding the electrode are altered.
Abiotic . Electromechanical failures include the breakage of the fine electrode shanks, degradation of insulation, and corrosion of metal contacts. These failures result in changes to an electrode’s exposed conductive surface area, conductivity, and, ultimately, its ability to transduce ionic extracellular signals into electrical signals ( ).
There are a wide breadth of neural recoding electrodes available, reflecting the huge motivation in the field to produce a device capable of long-term high-quality intracortical recordings and the diversity of targeted tissue regions for a variety of applications.
Commonly Used Electrodes for Intracortical Recording
There are a number of electrode designs used for cortical recordings, segmented here as: Utah arrays, Michigan-type electrodes, microwires, and encephalography (electrocorticography [ECoG] and electroencephalography [EEG]). The factors that influence the electrode selection primarily include invasiveness, spatial resolution, and relatedly quality and stability of the signal ( Fig. 28.2 ). To date, for neuroprosthetic BMI applications, the Utah and Michigan electrodes have outpaced microwires, ECoG and EEG, owing to their superior spatial resolution.

The Utah Electrode Array
The Utah Electrode Array (UEA) consists of silicon-based microelectrode typically with a 10 × 10 array of 1.5-mm tines ( ). Developed by Normann et al. at the University of Utah in 1991, it has remained at the forefront of the intracortical recording field. It provides extremely high-resolution information, with tines spaced 400 μm apart. Several challenges prevent its wider acceptance and use in broader applications: (1) it requires an invasive procedure, involving high-speed pneumatically driven insertion, and (2) it has suffered from inconsistent performance owing to biological (neuroinflammatory) response and/or manufacturing defects ( ). Nonetheless, the UEA is the only high-density, penetrating recording electrode approved by the FDA. It has 510(k) approval for recording or stimulation experiments of less than 30 days and investigational device exemption (IDE) approval for chronic recording or stimulation studies longer than 30 days ( ). Additionally, the Utah Array has received the CE mark in Europe.
Variations of the UEA have also been explored, including a slanted-tine design for achieving various tissue depths ( ), a design shape that conforms to complex anatomical geometries ( ), ultra-high aspect ratio devices ( ), and high-density arrays ( ). Recently, a collaboration between Brown University and Blackrock Microsystems has generated a commercially available wireless version of the UEA ( ).
The majority of clinical work with UEA technology to date has focused on rehabilitative applications ( ). As part of the BrainGate clinical trials, Hochberg et al. implanted UEAs into the primary motor cortex of their volunteers with tetraplegia. Using a robotic arm, they were able to restore reach and grasp functions to the individuals by recording and decoding volitional movement intent ( ). used similar approach to achieve virtual typing for paralyzed individuals, and demonstrated reaching and grasp through brain-controlled muscle stimulation. recently demonstrated neural recording for more than 1 year with UEAs implanted in patients with amyotrophic lateral sclerosis (ALS). Other groups around the globe have also been making significant advances in the field of BCI, including from Shwartz ( ), Andersen ( ), and Nicolelis ( ).
Despite the tremendous progress that has been achieved, improving recording performance and longevity remains a principal focus ( ). For examples, in their review of DARPA-led efforts in BCIs, argue that overcoming interface failure is one of the largest remaining hurdles for BCI researchers and that without overcoming these issues, the vision of life-long BCI systems will remain just out of reach.
Michigan-Style Microelectrodes
Whereas the Utah Arrays consist of a two-dimensional, 10 × 10 array of shanks, Michigan-style electrode arrays (MEAs) are planar devices with multiple recording contacts located along one or several silicon shanks ( ). Developed by Wise et al., first at Bell Labs in 1966 and later at the University of Michigan, these electrodes are frequently studied in preclinical BCI applications ( ). While chronic recording studies have been reported, the consistent longevity of recordings remains among the biggest hurdles ( ). To the authors’ knowledge, MEAs have yet to be studied in human clinical trials.
MEAs are sold commercially by NeuroNexus, a subsidiary of GreatBatch Inc. Recent advances have yielded on-board signal digitization (SmartProbe) and a variant that can be used as an optogenetic probe (optoelectrodes or simply “optrode”). For additional details on the development and application of MEAs, the reader is referred to a review by .
Microwires
Microwires were first developed by Salcman, Bak, and Schimdt at the NIH in the early 1970s ( ). These electrodes consisted of 2- to 11-μm-diameter iridium (Ir) and platinum (Pt) alloys. In one of the first long-term demonstrations of the technology, Salcman and Schmidt recorded single neuron unit activity for up to 223 days with a microwire implanted in the motor cortex of a nonhuman primate ( ). Since then, a variety of microwire devices have been developed for BMI applications ( ). However, as with other microelectrodes, neuroinflammation appears to degrade recording performance over time ( ), most likely through the degradation of insulating materials and corrosion of metals ( ).
Electrocorticography
ECoG is frequently used clinically to map epileptogenic regions of the brain and facilitate the surgical excision of operable focal lesions. ECoG arrays are placed temporarily intraoperatively and removed immediately or shortly after lesion resection surgery.
Many groups have investigated the use of ECoG arrays placed subdurally or epidurally to collect neural signals for use in BMI applications ( ). ECoG arrays pick up LFP signals and are reported to have a spatial resolution of about 4 mm ( ). Even with this limitation, applications involving two- and three-dimensional arm movements ( ) that predict arm movement with 3 degrees of freedom ( df ) ( ), and determine trajectory and kinetics for use in an FES system ( ) have been developed, with individual reports of chronic recordings up to 7 years ( ). While ECoG arrays have the distinct benefit of being placed superficial to the meninges, and thus potentially less invasive than implanted MEAs, most applications have focused on gross motor rather than fine motor tasks that may require a higher spatial resolution.
Electroencephalography
Due to noninvasiveness contacts placed on the scalp, EEG benefits from sampling a wider number of areas around the skull. However, its spatial resolution is relatively coarse at about 3 cm ( ). To date, the best performance of an EEG BCI system in control of extrinsic operations is 3 df , which was achieved only after months of intensive training ( ). Other BMI investigations include those by surveying the EEG response to various motor task ( ) and decoding movement intent in a sitting-to-standing task in healthy volunteers ( ). Interestingly, as one group has recently shown, EEG may be used in a complimentary fashion to other sources of neural control ( ): electrooculography in conjunction with EEG to improve hand-grasp task of an exoskeleton.
Optical Microelectrodes
Optical microelectrodes (or “optrodes”) are also under development by a number of groups, primarily for use in optogenetic research ( ). Notably, developed a micro-ECoG array that was capable of recording large-scale cortical activity spanning the S1 somatosensory and M1 motor regions in a nonhuman primate. With recent advances in optogentic techniques and novel implantable probe designs, such devices are improving at a rapid rate.
Summary of Intracortical Recording Electrode Failure Mechanisms
Failure of microelectrode recordings is characterized by a decrease in signal-to-noise ratio, leading to inability to accurately discriminate neuronal firing from background ( ). There are a number of underlying failure mechanisms that have been described, but, by definition, all lead to either electrical or neural circuit disruption. Failure modes for microelectrode recordings include:
- 1.
Degradation of insulation (e.g., dissolution, peeling of polymer coatings) ( )
- 2.
Corrosion of electrical contacts (e.g., oxidation, pitting of electrode contacts and connectors) ( )
- 3.
Local neurodegeneration (e.g., neuronal loss, dysfunction) ( )
- 4.
Device migration (e.g., movement of microelectrode away from neuron population of interest) ( )
- 5.
Direct mechanical damage (e.g., electrode, lead fractures), ( )
As suggested in Fig. 28.3 , the primary mechanisms are influenced by a number of secondary and tertiary factors, namely:
- •
Secondary (neuroinflammation, tissue strain, chemical environment, material fatigue)
- •
Tertiary (acute implantation injury, chronic micromotion and exposure, and manufacturing defects)

These factors are not mutually exclusive and may contribute synergistically to failure. In particular, it seems that neuroinflammation may underpin a number of interrelated failure mechanisms ( ). Neuroinflammation releases a number of cytotoxic substances (leading to neurodegeneration), altering the microenvironment by lowering pH and releasing reactive oxidative species (leading to corrosion and degradation of insulation), and depositing scar tissue (electrically and physically isolating the device from neurons).
Neuroinflammatory Underpinnings
While the dominant mechanism leading to functional loss following intracortical implantation is unresolved, neuroinflammation is thought to play a major role in both biotic and abiotic failure mechanisms ( ). Upon implantation, microelectrodes immediately disrupt brain tissue and neurovasculature, initiating a multiphasic inflammatory response ( ). The acute response plateaus within the first few weeks of implantation, and inflammation thereafter is driven in a chronic state via various mechanisms, including microglial and macrophage activation, the fibrotic glial scar, free radical oxidation, and chronic dysfunction of the blood–brain barrier (BBB) ( ).
To directly demonstrate the impact of neuroinflammation on recording signal quality, Harris et al. administered LPS (lipopolysaccharide, an endotoxin that elicits an inflammatory response) to rats and observed signal degradation within the first 4 weeks ( ). Signal degradation was observed as an increase in signal-to-noise ratio a decrease in local field potential power in the LPS-treated group compared with their control group. These results demonstrated that the inflammatory response directly affected signal quality during chronic recordings.
While the inflammatory process has been implicated in recording signal loss, so far no strategies to mitigate it have proved to be safe and effective for long-term use. A deeper understanding of the underlying biology is of significant importance. To better understand how to modulate/mitigate the inflammatory response, one must better understand the underlying cellular and physiologic changes that take place after implantation.
The Biological Response to Electrode Implantation
Overview
Upon implantation, the microelectrode disturbs the natural resting physiology of the brain tissue. Several groups have shown that the vasculature is immediately and significantly affected ( ). Small and large vessels may be disrupted, which allow cells, large proteins, and molecules that normally cannot pass through the BBB to pass into the extravascular tissue. Consequently, an inflammatory and wound-healing response is simultaneously set into motion ( ).
In cases of acute and nonsevere instances of brain injury, the BBB typically reforms after 3–4 weeks ( ), and weeks later, the inflammatory response subsides ( ). However, in the case of an implanted material, macrophages and microglia, the cells responsible for clearing debris ( ), are unable to engulf and phagocytose the microelectrode and continue to promote a proinflammatory state. Therefore, the blood–brain barrier remains chronically leaky ( ). Microglia and astrocytes begin to wall off the electrode, releasing reactive chemical species and depositing extracellular matrix proteins in a process known as astrogliosis. Astrogliosis results in fibrotic encapsulation around the implantation site.
In addition to the inflammation response set off by the initial injury, additional mechanisms, such as motion-induced injury, may promote persistent or chronic inflammation. Since the base materials in traditional electrodes are stiff (e.g., silicon), and the brain is comparatively much softer and in constant motion, albeit micromotion, chronic fluctuating strains are placed on the brain tissue surrounding the implanted electrode ( ). While the exact underlying biological mechanism for how the strain field is translated into inflammatory response is unknown, in vivo findings support the correlation. When compliant, rather than stiff, electrodes are implanted to minimize the strain/stress in response to motion, a reduced inflammatory response is observed in the rat brain tissue surrounding the electrode ( ).
Soluble Factors Kill Neurons, Degrade and Corrode Implanted Materials, and Maintain the Inflammatory Response
Soluble factors (e.g., growth factors and cytokines) play a major role in cell signaling to help mediate the wound healing response as well as inflammation after injury ( ). Upon initial injury, the BBB is disrupted, allowing macrophage, serum proteins, and other blood cells to infiltrate the immune-privileged neural space. In particular, macrophages and microglia have been identified as releasing a number of soluble factors that not only act on the tissue immediately touching the electrode but also diffuse from the implant surface, increasing the radius of the cells’ effects ( ).
Interleukins (tumor necrosis factor [TNF]α, interleukin [IL]-1, IL-6) are upregulated at the microelectrode location ( ). MCP-1, a chemoattractant, and metalloproteases (e.g., MMP-2, MMP-9) are also expressed ( ). TNFα, in particular, has direct cytotoxic effects on neurons, while both TNFα and MCP-1 have been shown to associate with the BBB and to help to recruit macrophages ( ). Proteases, among other roles, aid in breaking down extracellular matrix proteins. Further, reactive oxygen species appear to accumulate at the implant site ( ). Together, these soluble factors may have multiple effects, including material corrosion, degradation, and neuronal loss ( ). Importantly, reactive oxygen species and the other factors appear to further afflict the BBB, causing it to be “leaky” and allowing for additional cellular and serum protein infiltration, thus perpetuating an inflammatory cycle ( ).
Insoluble Factors Serve a Vital Role in Healing and Regeneration but Also Isolate Neurons Electrically and Physically From the Recording Electrode
Extracellular matrix proteins serve an important role after central nervous system injury: reconstruction and walling off the damaged tissue to prevent dysfunction and promote reformation of the BBB ( ). While the “dogma” in the field has historically categorized astrocytes as a primary cell type responsible for scarring and for walling off the injury site, new evidence strongly supports its role additionally as both one of regeneration and mediation of the inflammatory response ( ). After injury, glial fibrillary acidic protein (GFAP) and a number of proteoglycans (e.g., chondroitin sulfate [CSPGs]) are upregulated and expressed at the site of the electrode ( ). CSPGs are typically considered neuroinhibitory because they form synaptic-like connections with axons, entrapping neurons. However, it has been suggested that CSPGs may also serve a protective role by preventing neuronal destruction from invading macrophages ( ).
While it may be debated exactly how and what functions these molecules serve, their presence appears to displace neurons as well as change the electrical properties of the surrounding tissue, potentially limiting recording function ( ).
Neuronal Loss at the Electrode–Tissue Interface Attenuates the Source Signal
Neuronal density is significantly reduced surrounding implanted electrodes as a result of inflammation and fibrous scar tissue formation ( ). Given that electric field potentials decay rapidly, as an inverse square of distance from the source, proximity is an extremely important factor for recording electrode performance. Effective recording ranges have been estimated at 50–150 μm for local field potentials and 25–50 μm for single-unit recordings ( ). Unfortunately, neuron survival is not simply a function of the initial injury. Chronic inflammation also appears to reduce neuron populations; however, the exact relationship with recording quality has yet to be identified. Neuron viability is likely more relevant to recording quality than is the density. Regardless, as neurons die back, and are physically separated from the recording electrode by scar tissue, recorded signals are significantly attenuated ( ).
Intracortical Electrode Technologies to Evade the Inflammatory Response and Improve Long-Term Performance
Various strategies, specifically aimed at reducing the neuroinflammatory response, have been investigated to achieve recordings over clinically meaningful time frames. The list of strategies broadly include (1) drug administration, (2) material selection/modification, and (3) implantation technique/system design. The following sections and Fig. 28.4 serve as a primer for more in-depth reading on each approach.

Drug Administration
Drug administration is appealing because its effects are temporary and can be tuned to an individual’s needs. One must consider the safety, efficacy, ability of the drug to cross the BBB, and duration of a drug regimen as well as the desired effect. Some therapies have proved to be effective in the acute phase after implantation but due to safety concerns are not appropriate or practical for long-term use.
Broad-Spectrum Anti-Inflammatory Agents
Local anti-inflammatory treatment after implantation have been shown to reduce histological inflammatory response (dexamethasone) and improve recorded signal quality (minocycline), while vehicle control agents did not ( ). While anti-inflammatory drugs helps to confirm the role of inflammation, unfortunately, due to side effects of these therapeutics (i.e., osteoporosis, infection, cataracts, high blood pressure, impaired wound healing), chronic administration is contraindicated ( ). Minocycline is a tetracycline and appears to shift macrophages and microglia toward a quiescent, rather than activated, phenotype ( ). Promising in vivo data showed that neural recording from rats receiving minocycline fared better in signal-to-noise ratio and number of simultaneously viable channels. While recording was not investigated, dexamethasone reduced the histologically measured inflammatory response compared with untreated animals ( ).
Targeted Anti-Inflammatory Agents
The inflammatory response is extraordinarily complex and composed of many intertwined pathways ( ). Targeted agents may have the ability to more specifically address neuroinflammation in response to device implantation, without inducing the plethora of unwanted side effects seen with broad-spectrum anti-inflammatory agents. For example, α-melanocyte–stimulating hormone (α-MSH) and IL-1 receptor antagonist (IL-1Ra) are among the agents that have been tested for this purpose ( ).
α-MSH is an endogenous tridecapeptide that acts through the inhibition of proinflammatory cytokines and by inhibiting nitric oxide (NO) production ( ). By immobilizing α-MSH on a device both in vitro and in vivo, the Bellamkonda laboratory demonstrated a significant reduction in reactive astrocyte (GFAP) and macrophage/microglia (ED1) staining at both 1 and 4 weeks postimplantation ( ). investigated the antagonization of the proinflammatory cytokine IL-1Ra–coated microelectrodes. Short-term histologic studies verified IL-1Ra–coated microelectrodes significantly reduced astrogliosis compared with noncoated microelectrodes. Recently, showed that glial cell attachment to the electrode surface was significantly reduced in vitro with IL-1Ra incorporated in a PEG-based on-demand release system. While their in vivo study in rats produced less resounding results, they found that IgG, a marker for blood proteins, was significantly reduced in their IL-1Ra–positive group at 4 weeks after implantation. For both these targeted agents (α-MSH and IL-1Ra), longer-term histologic and recording outcomes have not been investigated and warrant additional research.
Drug/Coating Combinations
Antioxidants
Given the reported accumulation of reactive oxygen species at the implant site, researchers have investigated the use of antioxidants to combat their negative effects ( ). While there are a number of antioxidant agents readily available, a few of the most frequently investigated for use in central nervous system injury associated with microelectrode insertion include resveratrol, curcumin, and superoxide dismutase (SOD) mimetics [e.g., Mn(III) tetrakis(4-benzoic acid)porphyrin (MnTBAP)] ( ).
found that rats receiving resveratrol showed signs of improved BBB stability and increased neuronal density after 2, but not after 4, weeks at the microelectrode–tissue interface. While these effects were lost at 4 weeks, the authors suggest that the effects may have subsided as it was a single-dose experiment. They later tested repeated intraperitoneal administration and showed continued effects up to 16 weeks and, importantly, a reduction in neurodegeneration by staining with Fluoro-jade C ( ).
Surface modification with covalently bound antioxidative catalysts is appealing because the effects are targeted directly at the site of desired action. Additionally, as opposed to local drug delivery, where the bioactive molecule is consumed over time, permanent surface modification may have longer-lasting effects. For an initial proof of concept, Potter et al. coated a microelectrode surface with the SOD mimetic MnTBAP ( ). With this system, they observed a burst release of the molecule, accompanied with a sustained surface immobilized approach. The modified surfaces provided sustained activity, reducing the accumulation of reactive oxygen species up to several days ( ). Long-term histologic and recording outcomes are ongoing.
Neurotrophic Factors
To promote neural growth (neurite extension) toward the electrode surface, as well as to promote cell survival, several groups have investigated the local delivery of neurotrophic factors from electrode coatings ( ). In both studies, nerve growth factor was used for its ability to encourage neurite extension and even attachment on the electrode surface. While initial results have been promising, chronic applications have not been studied to know if the effect persists for prolonged periods of time and in the presence of the chronic inflammatory response. Further, translation of such a technique may prove to be challenging given the high regulatory burden required for biologics and drug-device combinations.
Material Selection/Surface Properties, Coatings
Cell Adhesion Proteins
Two primary reasons exist for the desire to increase cell adhesion to the electrode surface. First, increasing tissue adherence has been suggested to minimize the micromotion between the implant and the tissue. To date, this phenomenon has been demonstrated only in silico ( ). Additionally, minimizing the distance between electrode contacts and viable neurons has been suggested to increase the signal amplitude to single-unit neural recordings ( ). Therefore, several groups have applied biomolecules to electrodes to promote cell adhesion. For example, both laminin ( ) and L1 (a transmembrane neuronal cell adhesion molecule) have been used ( ). Unlike laminin, L1 demonstrated an increase in both neuron cell bodies and neuronal filaments at the electrode–tissue interface. Neither approach has been shown to impact neural recording quality, to date.
Hydrogel and Other Nonfouling Surfaces
Immediately on implantation, proteins are adsorbed onto the material’s surface. Depending on the protein milieu that is attracted and how those proteins are altered (e.g., denatured by electrostatic interactions), various attachment sites are produced that may help determine the cellular and inflammatory response. Therefore, several groups have investigated methods to prevent cell adhesion to the microelectrode surface.
Garcia et al. developed silicon microelectrodes coated with hydrogels consisting of poly(I-isopropylacrylamide) (pNIPAm) and cross-linked with poly(ethylene glycol diacrylate) (PEG) ( ). A frequently used tactic in surface modification, the PEG chains inhibit protein adsorption ( ). While the coating was effective at preventing cell adherence, in vivo studies yielded persistent inflammation and lower neuronal density for both uncoated and coated microelectrodes after implantation up to 24 weeks in rat cortex ( ). Similar results were found for Parylene C coatings ( ) and additional hydrogel coatings for review ( ).
Silicon carbide (SiC) is an attractive material, given its long track record and documented biocompatibility in other biomedical applications, including orthopedic implants and coronary stents ( ). Recently, characterized amorphous SiC as a biomaterial for chronic neural implants in vivo, demonstrating that it was not dissimilar to standard silicon device in terms of its histologic response (NeuN, CD68, and DAPI). For a more through discussion of SiC as a biomaterial, readers are referred to . Overall, while the nonfouling approaches may minimize surface attachment of inflammatory cells, glial scarring and neuron loss are not significantly impacted.
Soluble Factor (Cytokine) Sinks
Soluble factors such as cytokines play a major role in promoting and perpetuating the proinflammatory state. To counteract these soluble factors, researchers have developed passive diffusion “sinks,” or porous surface coatings, which absorb and entrap these potentially harmful molecules ( ). While a significant amount of evidence suggests this may be a viable technology, since the hydrogels typically used as passive sinks are themselves soft, it should be caveated that mechanical compliance may confound the interpretation of results ( ). Additionally, the implementation of hydrogel coating create the added complexity of increasing the functional distance between electrode contacts and nearby neurons.
Conductive Polymers
To increase the biocompatibility of a material, researchers often look to mimic the native biological environment. While tissue conducts electricity via ionic transport, electronic devices conduct electrons. Conducting polymers complexed with biologically active counterions are unique in that they can act in a hybrid fashion, enabling either electron or ionic transport ( ). Further, they can be directly deposited onto microelectrode substrates, with relatively simple manufacturing processes ( ). It is unclear whether conductive polymers indeed increase the biocompatibility of devices as a result of their electrical conduction, or whether it is by other mechanisms, but a number of groups have reported success with poly(3,4-ethylenedioxythiophene) (PEDOT) coatings integrated in microelectrode devices ( ). Reports of the performance of PEDOT are exceedingly positive, with several recent reports of its inclusion in microelectrode recording applications ( ). However, some skepticism remains as to long-term stability of the polymers in vivo, especially for stimulating applications.
Material Selection/Bulk Properties
Flexible Substrates
A number of flexible microelectrode designs have been described in the literature including those made from polyimide, benzocyclobutene (BCB), polydimethylsiloxane (PDMS), Parylene C, and SU-8 ( ). With these, researchers have been able to successfully record neurons from rat cortex, BCB ( ), and even record single units, SU-8 ( ). Additionally, a number of innovative flexible probe designs have been recently published, including sinusoidal designs ( ) and for peripheral applications a flexural neural ribbon ( ). Excitingly, recently demonstrated an injectable mesh microelectrode. Giving more credence to the hypothesis that flexible electrodes may help to evade the inflammatory response, demonstrated robust cortical recording up to 16 weeks, with minimal inflammatory response, which they deemed “glial scar free.” They performed in vivo two-photon imaging and incredibly tracked the same neuronal population over the several-month study and showed that they survived in close proximity to the neural probe. To overcome the flexibility during insertion, they developed a simple introducer system consisting of a rod with a hook at the end that appears to drag the electrode through the tissue.
While flexible electrode designs show a significant amount of potential, to date, there have been no comprehensive systematic studies comparing recording performance and longevity between stiff and flexible electrodes controlling for all other variables, such as having similar surface chemistry. Further, one of the main drawbacks of an entirely flexible electrode is that it is often impossible to, by itself, be inserted into the brain without buckling.
Methods to address soft microelectrode insertion (namely prevention of buckling) include coating microelectrodes with sacrificial polymers or coatings that dissolve after insertion ( ), fast insertion speed ( ), treating implantation site with collagenase to soften the tissue prior to insertion ( ), a variety of introducer designs ( ), and materials that dynamically soften after insertion ( ). Each approach is at a different stage of development.
Dynamically Softening Materials
Dynamically softening materials used for intracortical microelectrodes were first introduced as a means to both enable the insertion of a less-rigid device and to minimize the effects of chronic micromotion and mechanical mismatch between the microelectrode and the tissue ( ). The first-generation mechanically responsive microelectrodes developed by Capadona et al. mimicked the sea cucumber, which reacts to threats by rapidly transitioning their outer shell from soft to stiff via chemically induced cross-linking. As an initial finding, the team demonstrated the ability of the dry implants to be easily inserted through the pia mater and into the brain cortex of a rat ( ). Histologic evaluations by demonstrated nearly significantly reduced inflammatory response and minimal loss of neuronal density by 16 weeks postimplantation. Additionally, Hess et al. have demonstrated several advances in fabrication techniques leading to the inclusion of a Parylene C coating for additional electric insulation between sputtered contacts ( ).
Alternatively to the sea cucumber inspired materials, a few laboratories have also explored the use of shape memory polymers (SMPs) for their tunable thermal and mechanical transitions ( ). For example, the Voit laboratory has developed SMP-based microelectrodes from acrylate and thiolene/acrylate polymers ( ). Initial studies demonstrated that their SMP-based microelectrodes were capable of recording neuronal signals in a rat cortex. However, the team has not yet completed their histologic or electrophysiologic head-to-head comparison to that of traditional microelectrodes ( ).
Nanomaterials/Carbon Nanotubes
Nanomaterials are an exciting class of materials that have been tested in a variety of biomedical applications, including for neural interfacing ( ). Notably, nanomaterials have the potential to interact with biological tissues at the cell and even subcellular levels, facilitate transportation of molecules, and have desirable electrical properties (conduct electrical/ionic charges) ( ).
Carbon nanotubes (CNTs), carbon nanofibers (CNFs), and graphene-based materials, due to their extraordinary strength and electrical conductivity and the number of attributes that can be designed into them, CNTs have been widely studied for use in microelectrodes ( ). However, despite these advantages, CNTs by themselves are rigid and non-compliant and tend to have high electrical impedance due to their extremely small dimensions.
To develop flexible nanotube systems, CNTs have been combined with a number of flexible substrates, including polyimide, PDMS, and Parylene C ( ). Further, to overcome the challenge of high impedance, several groups have investigated inclusion of PEDOT in their material matrices ( ). In one study, observed chronic spike recording stability up to at least 4 months. conjugated a bioactive peptide (YIGSR) to the CNT surface to promote cell adhesion. The pace and quality of developments in the field of nanomaterials for recording interfaces are rapid and exciting. When also considering new medical applications that include both electrical stimulation and recording, nanomaterials hold significant potential ( ).
Implantation Technique and System Design
Avoidance of Vasculature
Intracortical microelectrodes are invasive by nature, and inevitably damage occurs during surgical implantation. Live imaging of in vivo preparations has made it possible to observe the dynamic and longitudinal response to microelectrode insertion, particularly of the vasculature ( ). The impact on blood vessels is immediate and extensive and persists for a long duration. According to research by , the chronic breach in the BBB may play a major role in determining electrophysiologic performance. In the future, image-guided techniques such as those taken by may provide a means to minimize damage to the brain tissue during insertion, particularly the vasculature.
Shape and Speed of Insertion
Several groups have investigated electrode shapes and insertion speeds to minimize damage to the tissues ( ). suggested, based on computational modeling and ex vivo studies, the sharpest (5 degrees) and fastest (2 mm/s) insertion minimized potential for damage based on blood vessel displacement, rupture, severing and dragging, as well as overall tissue strain. Findings from Bjorssen et al. are consistent with techniques described by Rosuche et al. for extremely fast pneumatic insertion and, more recently, by Dryg et al. for magnetic insertion of microelectrodes ( ).
However, there are a number of other groups that have tested moderately fast insertion speeds ranging from 0.1 to 10 mm/s that suggest perhaps the opposite is true. Their findings suggest that speed may have less impact than tip shape. Specifically, Fekete et al. found that tip shape, namely its sharpness, played a significant role in the peak force of insertion. Fekete was able to reduce insertion force by nearly 5-fold with sharpening of the silicon probe alone ( ). Interestingly, in the same study, however, they found that increased speeds led to doubled insertion forces and produced minimal effect on dimpling ( ). These findings are echoed by , who observed minimal impact of the speed of insertion on neuroinflammatory histologic outcomes among the range of moderate insertion speeds. Therefore, it appears that there may be a more complex answer than simply faster is better, depending on whether one is trying to minimize shear stress, damage to vasculature, or dimpling during insertion. The optimal speed and tip shape appear to be dependent on each other and perhaps specific applications.
Floating Versus Tethered Lead Wires
Most electrode designs currently incorporate tethers cemented into the skull, which mechanically fixes the electrode at its base and therefore limits movement with the brain (particularly for stiff electrodes). Fixation to the skull contributes to a fluctuating strain within the brain tissue that may lead to tissue damage with chronic exposure ( ). Floating designs ( ) and designs with extreme flexural compliance ( ) may reduce the tethering effect. Given recent advances in devices with wireless communication capabilities and compliant materials, this challenge may be mitigated even further.
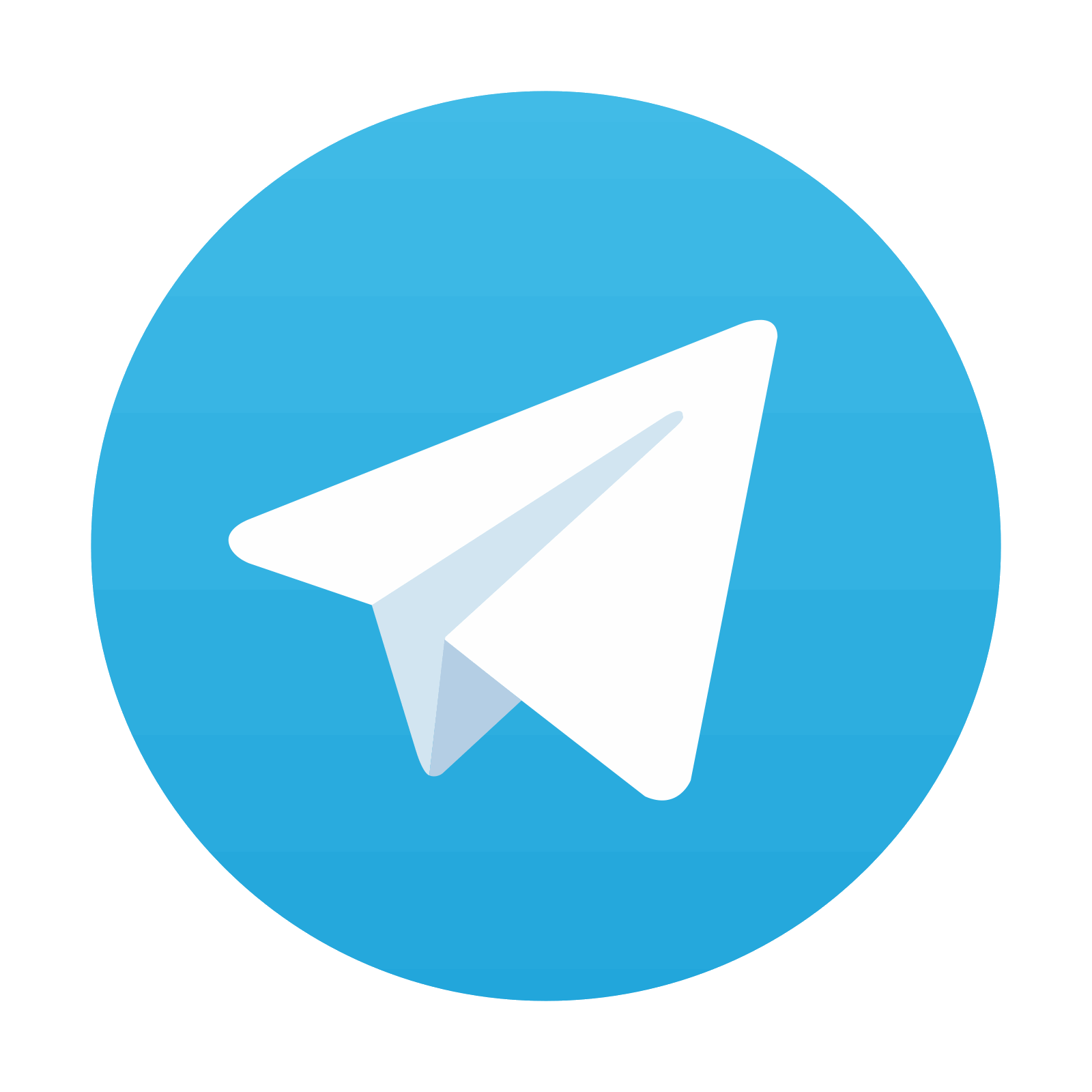
Stay updated, free articles. Join our Telegram channel
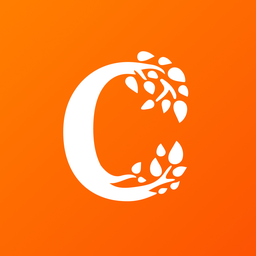
Full access? Get Clinical Tree
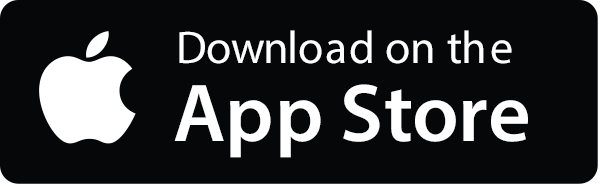
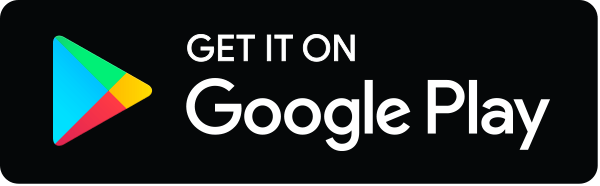