The term quantitative electromyography (QEMG) refers to a number of different electrodiagnostic techniques and methods of gathering and analyzing data ( Table 12-1 ). No simple definition applies to all aspects of QEMG. QEMG is distinguished from routine electrodiagnostic tests by the continuously variable nature of the data (cardinal data) and by the ability to make statistical inferences and comparisons. The quantitative nature of QEMG is in contrast to the subjective and relative-based data (ordinal data) obtained from most needle EMG studies. For example, data from routine needle EMG studies represent interpretations of motor unit action potential (MUAP) waveforms expressed in relative units based on arbitrary scales that do not have physiologic bases (up and down arrows and gradations noted by plus and minus symbols). In QEMG the same waveform data are expressed in physical and physiologic units (millivolts, milliseconds, milliseconds * millivolts, number of phases and turns), and the averages of such values from a muscle can be presented as summary data. QEMG relies heavily on computers that are integral to modern EMG machines. Computers also allow for the calculation of derived metrics (e.g., MUAP area and area/amplitude ratio, ratio of average number of waveform turns/waveform amplitude, and discharge–discharge waveform component variability). Data can also be viewed in unique displays. The continuously variable nature of QEMG data and its statistical representations (e.g., mean, median, and standard deviation) permit statistically valid comparisons between muscles within a patient and between patients, between serial studies within a patient, and between electrodiagnostic laboratories. Statistical analysis of QEMG data can be used as an expert system when combined with EMG database libraries to provide probabilities of a particular diagnosis.
QEMG Study | Electrode | Information |
---|---|---|
Individual MUAP analysis | Concentric or monopolar | Quantitative MUAP waveform metrics |
Automated MUAP analysis | Concentric or monopolar | Quantitative MUAP waveform metrics |
Fiber-density | Single-fiber | Muscle-fiber density |
Macro-EMG | Macro single-fiber | Whole motor unit size |
Scanning EMG | Concentric | Three-dimensional motor unit view |
Motor unit number estimation | Surface | Estimate of number of motor units innervating a muscle |
Neuromuscular variability | Single-fiber or small concentric | Quantitative neuromuscular variability (jitter) |
CMAP scan | Surface | Estimate of size and excitability of motor units |
Of historical note, most principles that underlie the qualitative interpretation of routine needle EMG MUAP waveforms are based on data from early QEMG studies by Buchthal and colleagues in the 1950s. These studies were performed with manual measurements from photographic records and were time-consuming; similar analyses can now be performed in seconds compared to hours, thus making QEMG practical.
The QEMG technique used most commonly is automated extraction of MUAPs. Some QEMG techniques rely on special electrodes ( Fig. 12-1 ). For routine studies, standard concentric and monopolar electrodes provide comparable information, but MUAP amplitude and duration values are greater with monopolar electrodes. For MUAP analysis, there are no significant differences between standard- and pediatric-sized concentric electrodes, but the pediatric-sized concentric electrode allows neuromuscular jitter to be measured with results similar to those obtained with a single-fiber electrode. Special electrodes include single-fiber electrodes for assessment of motor unit fiber density and the variability of neuromuscular junction transmission, macro-EMG electrodes to assess the size of the whole motor unit, and a device to slowly withdraw the electrode in scanning EMG to determine the spatial and temporal distribution of the motor unit within a muscle. Special algorithms include plotting of metrics from interference analysis and methods to estimate the number of motor units innervating a muscle by motor unit number estimation (MUNE).

The selection and use of the various QEMG techniques depend on the clinical question to which an answer is sought and on specific computer software programs. The advantage of QEMG is that it is objective. QEMG changes may precede changes observed with routine electrodiagnostic studies. QEMG can also help prevent false-positive interpretations, as the statistical approach places less emphasis on rare outlying waveforms and data that may be overweighted in interpretation during qualitative studies.
There are limitations and cautions regarding QEMG. Electrodiagnosis is a tool, and QEMG cannot “deliver” a diagnosis. Results must make sense in the clinical context. Excessive reliance on a numeric value, without considering a broad spectrum of clinical data, may lead to diagnostic errors with QEMG, as with routine electrodiagnostic studies. Most importantly, many QEMG techniques rely on computer-based analysis with waveform markings and values derived automatically from software algorithms. Marking algorithms can result in marking errors, and all QEMG data must be verified by inspection, and errors corrected. Despite the speed of data acquisition, QEMG takes time, perhaps more than routine electrodiagnostic testing, and is not a shortcut to the diagnosis. These issues notwithstanding, certain aspects of QEMG can be incorporated easily into routine studies to enhance the diagnostic process.
Motor unit action potential analysis
QEMG of MUAPs requires isolation of individual MUAPs from the interference pattern and marking of various portions of the waveforms. Isolation may be accomplished by manual or automated methods. Similarly, marking may be performed manually, but more commonly it is performed automatically, with the ability to make changes.
Manual Motor Unit Isolation
During routine EMG recording (with concentric or monopolar electrodes), a low voluntary effort generates a weak interference pattern with three or four well-defined MUAPs on the screen and a number of less defined motor units in the background ( Fig. 12-2 ). The discharge pattern is viewed in “free run” or in continuous sweep mode, and the sweep speed usually is set to 10 msec/div, resulting in MUAPs that are compressed visually. Motor units discharge independently of each other, resulting in occasional superimposition of MUAPs. Under these conditions, accurate estimates of amplitude, duration, and complexity (phases and turns) are difficult, and only qualitative assessments of these metrics can be made; small MUAP changes are unlikely to be recognized.

Individual MUAPs can be isolated for more accurate analysis. Historically, MUAPs were captured on photographic film, which is very time-consuming and is not feasible for clinical application. Individual MUAPs can be isolated more easily for detailed and accurate observation by the use of a voltage trigger and delay line. With this procedure, the needle electrode is adjusted so that one MUAP has a greater peak amplitude than the others. The trigger voltage level is set so that a negative- or positive-going portion of this MUAP crosses the set voltage level (see Fig. 12-2 ). The triggered MUAP is viewed on another display screen every time it discharges. This ensures that the same MUAP is captured every time. Triggered motor units may be frozen or averaged to eliminate contamination by background (distant) MUAPs. The sweep speed can be expanded to 2 to 5 msec/div to view MUAPs in greater detail, and quantitative measurements can be made on the isolated MUAPs. The needle then is moved to isolate another MUAP, and the process is repeated.
The delay line takes advantage of the computer-based design of modern EMG machines. Analog waveform signals are converted to digital signals and stored in a short-term memory module. The short-term memory stores a limited duration of the signal, and incoming waveform data displace old data. At the time the waveform crosses the trigger voltage, the computer looks back in time to display the whole MUAP waveform (see Fig. 12-2 ).
The trigger and delay line can be used easily during routine needle EMG studies in any muscle to obtain detailed views of MUAP waveforms. After obtaining basic data on recruitment and amplitude from the free-run mode, 10 to 20 triggered MUAP waveforms are observed objectively (but qualitatively) at expanded sweep speeds to verify normality or detect subtle pathologic changes such as increased number of turns. A decision then is made about whether to perform QEMG. During examination of triggered MUAP waveforms, the stability of late components can be assessed when successive waveforms are superimposed ( Fig. 12-3 ). Instability viewed in this way represents abnormal transmission at several neuromuscular junctions and is called “jiggle.” MUAP instability can represent pathology from reinnervation in denervating disorders or slowed muscle fiber conduction velocities in both denervating and myopathic disorders. With an increase in the low-frequency filter to 1,000 Hz and use of a pediatric-sized electrode or small monopolar electrode, formal measurement of neuromuscular jitter can be made, and will be discussed later.

Automated Motor Unit Isolation
The computer capabilities of EMG machines can be used to extract more MUAPs than by the manual, one-at-a-time method using the trigger and delay line. Automated systems have been developed to identify and measure a number of MUAPs from the same interference pattern.
The digitized interference pattern is analyzed by detection algorithms that can identify individual MUAP waveforms ( Fig. 12-4 ). A variety of algorithms have been developed based on waveform template matching and decomposition; these are available on many EMG machines. Several terms have been given to the automated process, including decomposition EMG, multi-motor unit potential (MUP) analysis , and multi-MUP analysis . There have been few comparisons of the accuracy of MUAP extraction algorithms, and not all perform equally.

MUAP data obtained by isolating single MUAPs differ from those obtained by automated systems ( Table 12-2 ). With the former technique, low-threshold motor units are isolated by adjusting the electrode position to achieve a main spike rise-time of 0.5 to 1 msec, thus optimizing MUAP amplitude. Summary statistics are derived from approximately 20 MUAPs, with no appreciable changes in metric values when more MUAPs are collected. With the automated technique, a more vigorous interference pattern is generated and higher-threshold motor units can be isolated. One automated QEMG program is able to record the surface activity of the muscle under study with a second surface electrode as a root-mean-square signal, which correlates linearly with force. By keeping the electrical interference pattern activity within a narrow zone, QEMG data are more consistent. Within the interference pattern, only one or two of the isolated MUAPs have short rise-times and optimized amplitudes, whereas the other extracted MUAPs have longer rise-times and lower amplitudes. Accordingly, the populations of MUAPs differ between the manual and automated techniques. Because of these differences, it is important to obtain reference values in each laboratory for a number of index muscles.
Film | Trigger/Delay Line | Automated | |
---|---|---|---|
Selection of MUAPs | Manual | Manual | Automated |
Investigator bias | Yes | Yes | Minor |
Amplitude bias | Above 50 μV | Highest | Above 50 μV |
Contraction level | Low | Low | Low–moderate |
Time resolution | Low | High | High |
Number MUAPs/site | 1–2 | 1 | 4–8 |
Analysis time | ~1 hr | ~20 min | ~5 min |
MUAP Metrics
MUAPs isolated by either method must be marked accurately for reliable data. With the manual system, isolated motor unit waveforms may be marked by the operator for duration, amplitude, and number of phases and turns. More commonly, isolated MUAPs are marked automatically by the computer using marking algorithms. Detection of peak amplitudes and baseline crossing is straightforward; however, algorithms for marking MUAP onset and termination points and turns differ among EMG machines ( Fig. 12-5 ). During algorithm development, the various parameters within the algorithm are adjusted until the resultant marks match those set manually by experienced electromyographers. Little information about the marking algorithms accompanies EMG machines, and there is no ability to change marking parameters. Comparisons between EMG machines and their marking algorithms for MUAP duration show clinically significant differences, including those in other metrics that are based on duration, such as area and area/amplitude ratio. MUAP duration also affects how many phases are counted. Accordingly, it is most important to review each isolated MUAP and adjust markers as necessary.

The MUAP waveform can be analyzed and characterized by a number of metrics, including those based on physiologic features and derived features ( Fig. 12-6 ). The goal in selecting among various metrics is to distinguish normal from abnormal and, among abnormal, between neuropathic and myopathic disorders. However, only a relatively small number of MUAPs in a diseased muscle have abnormal waveforms and metrics, and there is overlap among traditional metrics of amplitude, duration, and number of phases between normal, neuropathic, and myopathic muscles ( Fig. 12-7 ). Although MUAP duration traditionally has been used to distinguish between neurogenic and myopathic motor units, determination of the onset and, particularly, the termination of the waveform is problematic. Waveform marking depends on display sensitivity, baseline noise, and marking algorithms. Efforts have been made to develop metrics that better distinguish between these pathologic states and normal. The ratio of the rectified area to the peak-to-peak amplitude has been found to have the least overlap and best discrimination. Another approach is to define the range of normal metrics statistically and then assess a muscle to determine whether, among the 20 MUAPs studied, a sufficient number (percentage) of MUAPs have outlying metrics to indicate presence and type of abnormality.


A quite different approach involves pattern discovery in the muscle under investigation based on comparisons with patterns of MUAP waveform features recorded from normal, neuropathic, and myopathic muscles, the latter two confirmed by pathology or genotyping. The pattern discovery is accomplished using Bayesian methods to characterize the muscle. This can be used as a decision support aid for QEMG by providing probabilities that a muscle is normal or abnormal and the underlying pathology.
Automated QEMG is available on most commercial EMG machines, although the software may be offered as an option. QEMG can be applied readily during routine EMG studies, and is useful to help distinguish between normal and myopathic states, especially when clinical symptoms and signs are subtle. Familiarity with the particular technique is essential, as is the gathering of reference data from normal subjects in an index muscle. For the analysis of a myopathy, the biceps-brachialis muscle is a reasonable choice for an index muscle because the subject can exert a controlled contraction and it is a proximal muscle that usually is involved. Twenty or more MUAPs can be collected readily and inspected for marking accuracy. The summary statistics for amplitude, duration, area/amplitude ratio, and number of phases and turns can be compared with normal values.
Interference pattern analysis
The QEMG methods described above refer to isolation and measurement of individual MUAPs. This must be carried out at low levels of contraction when individual potentials can be isolated and therefore may reflect a biased sample of MUAPs activated at the low end of the recruitment spectrum rather than an estimate of the full spectrum of MUAPs. Analysis of the interference pattern over a range of force levels was developed to discern rapid recruitment of complex MUAPs in myopathic disorders, and in particular to provide a quantitative way to identify MUAP abnormalities when they are of mild degree. Early efforts were semiautomated, with analysis performed offline, and provided data on the number of turns in the interference pattern and the mean amplitude between turns at different levels of force. Later efforts, aided by increasing use of digital computers, explored other metrics, such as integration or spectral analysis of the interference pattern, number of baseline crossings, discharge intervals, and assessment at set levels (forces) of contraction. A robust approach is based on plotting turns/second against mean amplitude/turn at different force levels over the full range of force generation in normal muscle, resulting in a “cloud” of points ( Fig. 12-8 ). Clouds differ with gender, age, muscles, and types of EMG electrodes (concentric or monopolar), and confidence limits enclosing the clouds were derived from these combinations. Abnormal myopathic clouds are defined as more than 10 percent of 20 points below the normal cloud; abnormal neuropathic clouds have more than 10 percent of 20 points above the normal cloud. Later efforts used simulation data to construct interference patterns to identify unique metrics, but such measurements in clinical practice require special software, restricting their use.

Single-fiber EMG
The term single-fiber EMG (SF-EMG) refers to a number of electrodiagnostic techniques that rely on the use of a special needle electrode that has a very small recording surface located on the side of the cannula (see Fig. 12-1 ). The short recording radius (300 μm) of the electrode allows it to record single muscle fiber action potentials. Single-fiber electrodes can be used to collect several types of data: (1) variability of neuromuscular junction transmission at the level of a single junction or pairs of junctions; (2) fiber density—namely, the relative density of muscle fibers within a motor unit; and (3) macro-EMG, the MUAP representing activity of all muscle fibers in the motor unit by using a modification to a single-fiber electrode. Single-fiber electrodes are expensive but reusable. A special cable is required. Electrodes must be refurbished after 20 or so uses by sharpening the beveled tip and etching the recording surface.
Neuromuscular Jitter
The discharge-to-discharge variability inherent in transmission across the neuromuscular junction can be assessed when single-fiber action potentials are recorded. There are two techniques to activate transmission across the junction: one is to stimulate intramuscular nerve branches and record from single muscle fibers (called stimulation SF-EMG), and the other is to have the patient voluntarily activate motor units and record from pairs of muscle fibers from the same motor unit (called voluntary SF-EMG). A degree of transmission variability is normal, and is called “jitter.” Variability relates to several factors: (1) the range of conduction velocities along nerve terminal branches of varying length; (2) time differences in presynaptic mobilization and release of acetylcholine; (3) time differences in opening of postsynaptic acetylcholine receptors; and (4) the threshold for opening voltage-gated sodium channels. Normal jitter values are in the range of microseconds, are determined empirically, and differ by age and among muscles. Excess variability reflects altered transmission, but is not specific for site of pathology (presynaptic or postsynaptic), and can be due to abnormalities in any one or a combination of the above sites. SF-EMG recording of jitter is discussed in detail in Chapter 17 , but techniques that assess jitter using concentric or monopolar electrodes will be reviewed here.
Fiber Density
The arrangement of muscle fibers within a normal motor unit is not uniform, and few fibers of the same motor unit are contiguous. The number of muscle fibers recorded as a MUAP by an intramuscular electrode depends on the uptake radius of the electrode. Concentric and monopolar EMG needle electrodes have an uptake radius of approximately 1,500 μm and record activity from 7 to 15 fibers of a motor unit (see Fig. 12-1 ). Single-fiber needle electrodes have a restricted recording uptake radius of approximately 300 μm and record activity from 1 to 3 fibers. The number of fibers in a normal motor unit varies among muscles, but it is estimated to range from 100 to 600 in muscles commonly studied. The routine MUAP waveform therefore reflects less than 10 percent of the total number of fibers of the motor unit. The single-fiber electrode offers a very restricted but detailed view. Advantage can be taken of the short recording radius of the single-fiber electrode to detect small changes in the density of muscle fibers within a motor unit, which thus is a sensitive measure of altered muscle architecture.
The configuration of a single-fiber electrode includes a 0.003-mm 2 active recording surface located in a side port approximately 5 mm from the beveled tip (see Fig. 12-1 ). The cannula serves as the reference electrode. Fiber density measurements are straightforward to perform on any EMG machine with a trigger and delay line. The low filter is set to 500 Hz, the sweep to 1 msec/div, and the display sensitivity at 100 μV/div. The procedure is to isolate a single-fiber action potential during weak voluntary activation. As an aid to isolation, the low-filter setting is raised to 500 Hz to reduce background slow-wave activity from distant muscle fibers. A voltage trigger and delay line are used to verify that the same index single-fiber action potential is observed from discharge to discharge. To ensure that the recording electrode is reasonably close to the index muscle fiber, the waveform rise-time must be less than 300 μsec and the amplitude greater than 200 μV. When the triggered waveform is stable over approximately 20 sweeps, the waveform is inspected to determine whether other potentials or components are associated with the index potential. Discrete inflections are counted as additional fibers ( Fig. 12-9 ). The number of associated potentials reflects the fiber density of that motor unit. The electrode is moved and another index potential is isolated, and the average of 20 observations is used for the fiber-density value of the muscle.

Fiber density values reflect empiric data on the distribution of muscle fibers within motor units. Any muscle can be studied. Values vary among muscles and increase with age above 65 years, and this has been attributed to subclinical, age-related motor neuron loss (see Fig. 12-9 ). Tables of normal values reflect 95 percent confidence limits, and averaged values in a muscle that exceed these limits represent pathology ( Table 12-3 ).
1–10 yr | 11–20 yr | 21–30 yr | 31–40 yr | 41–50 yr | 51–60 yr | 61–70 yr | 71–80 yr | 81–90 yr | |
---|---|---|---|---|---|---|---|---|---|
Frontalis | 1.67 | 1.67 | 1.68 | 1.69 | 1.70 | 1.73 | 1.76 | ||
Tongue | 1.78 | 1.78 | 1.78 | 1.78 | 1.78 | 1.79 | 1.79 | ||
SCM | 1.89 | 1.86 | 1.90 | 1.92 | 1.96 | 2.01 | 2.08 | ||
Deltoid | 1.56 | 1.56 | 1.57 | 1.57 | 1.58 | 1.56 | 1.60 | 1.62 | 1.65 |
Biceps | 1.52 | 1.52 | 1.53 | 1.54 | 1.57 | 1.60 | 1.65 | 1.72 | 1.80 |
EDC | 1.77 | 1.78 | 1.80 | 1.83 | 1.90 | 1.99 | 2.12 | 2.29 | 2.51 |
ADQ | 1.99 | 2.00 | 2.03 | 2.08 | 2.16 | 2.28 | 2.46 | ||
Quadriceps | 1.93 | 1.94 | 1.96 | 1.99 | 2.05 | 2.14 | 2.26 | 2.43 | |
Anterior tibial | 1.94 | 1.94 | 1.96 | 1.98 | 2.02 | 2.07 | 2.15 | 2.26 | |
Soleus | 1.56 | 1.56 | 1.56 | 1.57 | 1.56 | 1.62 | 1.66 | 1.71 |
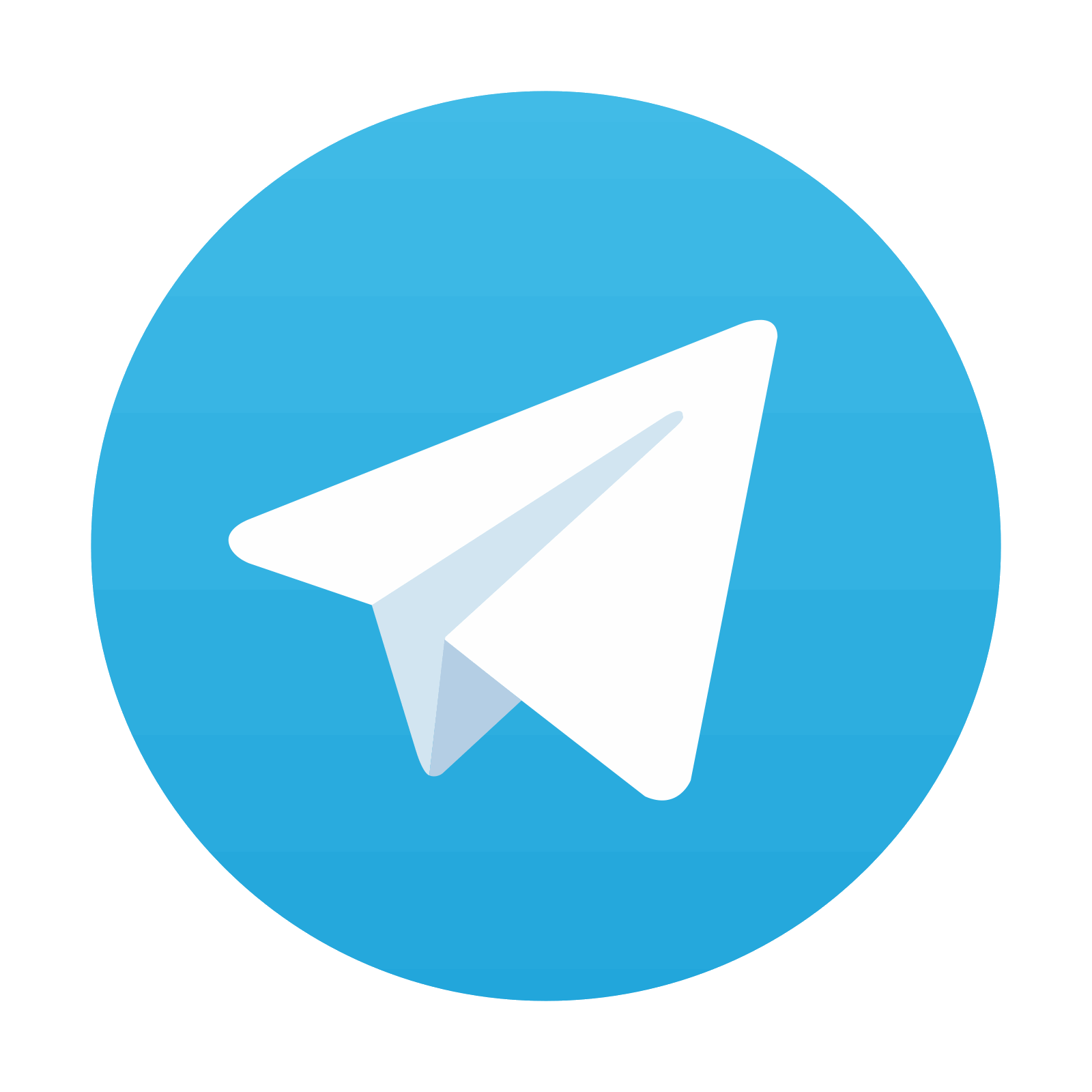
Stay updated, free articles. Join our Telegram channel
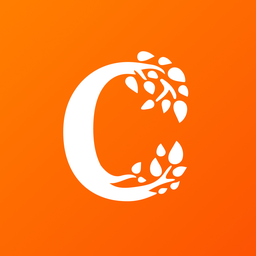
Full access? Get Clinical Tree
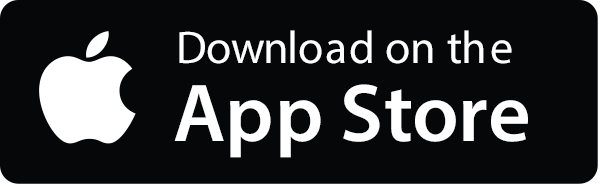
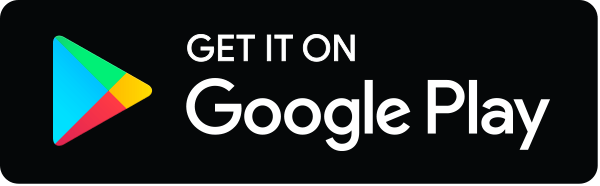
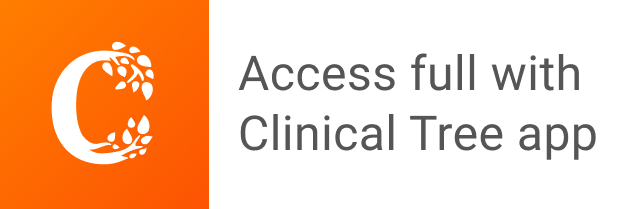