Abstract
Highly useful hearing, including speech reception without any visual cues, can be reinstated or produced for the first time with a cochlear implant—a device that bypasses damaged or missing sensory hair cells in the cochleas of deaf or severely hearing-impaired persons using direct electrical activation of remaining auditory neurons. As such, it is the first substantial restoration of a human sense using a medical intervention, and is a triumph of otology, neuroscience, and biomedical engineering among other fields. This chapter describes how the implant works and possibilities for future improvements.
Keywords
Auditory nerve, Auditory prosthesis, Cochlea, Cochlear implant, Deafness, Neural prosthesis
Outline
Design of Cochlear Implants 1209
Aspects of Normal Hearing 1209
Loss of Hearing 1210
Electrical Stimulation of the Auditory Nerve 1210
Components of Cochlear Implant Systems 1213
Transformation of Input Sounds Into Stimuli for the Implant 1214
Performance With the Present-Day Unilateral Implants 1215
Adjunctive Stimulation 1218
Possibilities for the Future 1219
Acknowledgments [CR]
References 1219
Acknowledgments
This chapter is an update of the chapter with the same title in the first edition of this book. Parts of the update were drawn from several recent publications, including and . Author BSW is a consultant for MED EL GmbH and author MFD is a consultant for Advanced Bionics LLC. However, none of the statements in this chapter favors either of those companies or any other company.
Deafness and severe impairments in hearing were hopeless conditions until only recently. Controlled electrical stimulation of the auditory nerve changed that, and the resulting return of hearing is now widely regarded as one of the great advances in modern medicine.
The device that produces and presents the electrical stimuli is called a cochlear implant (CI). Just 40 years ago CIs provided little more than a sensation of sound and sound cadences ( ). The implants were useful for an alerting function (e.g., hearing a loud sound that might be an oncoming car) and as an adjunct to lipreading. Many experts in otology and the hearing sciences at the time were highly skeptical that implants could ever support useful speech reception with hearing alone. In the 1980s, however, implant systems with multiple channels of sound processing and multiple sites of stimulation in the cochlea were developed, and these systems supported significantly higher levels of speech reception than their single-channel and single-site predecessors. In the late 1980s and continuing to the present, new and better processing strategies, in conjunction with multielectrode implants, have produced additional large gains in performance. Indeed, a principal conclusion of the 1995 United States National Institutes of Health (NIH) Consensus Conference on Cochlear Implants in Adults and Children ( ) was that “A majority of those individuals with the latest speech processors for their implants will score above 80 percent correct on high-context sentences, even without visual cues.” This degree of functional restoration is remarkable, and far greater than that achieved to date with any other type of neural prosthesis.
More about the history of the CI is presented in Chapter 99 in this book.
The primary purpose of the present chapter is to indicate how electrical stimulation at the auditory nerve can bring a person from total or nearly total deafness to useful hearing. In addition, some possibilities for further improvements in the design and performance of CIs are mentioned. Further information about these topics is presented in several detailed reviews published during the past 10 years, including , and .
Design of Cochlear Implants
Aspects of Normal Hearing
In normal hearing sound waves traveling through air reach the tympanic membrane via the ear canal, causing vibrations that move the three small bones of the middle ear. This action produces a piston-like movement of the stapes, the third bone in the chain. The “footplate” of the stapes is attached to a flexible membrane in the bony shell of the cochlea called the oval window. Inward and outward movements of this membrane induce pressure oscillations in the cochlear fluids, which in turn initiate a traveling wave of displacement along the basilar membrane (BM), a highly specialized structure that divides the cochlea along its length. This membrane has graded mechanical properties. At the base of the cochlea, near the stapes and the oval window, it is narrow and stiff. At the other end, near the apex, the membrane is wide and flexible. These properties give rise to the traveling wave and to points of maximal response according to the frequency or frequencies of the pressure oscillations in the cochlear fluids. The traveling wave propagates from the base to the apex. For an oscillation with a single frequency, the magnitude of displacements increases up to a particular point along the membrane and then drops precipitously thereafter. High frequencies produce maxima near the base of the cochlea, whereas low frequencies produce maxima near the apex.
Motion of the BM is sensed by the inner hair cells (IHCs) in the cochlea, which are attached to the top of the BM in a matrix of cells called the organ of Corti. Each hair cell has fine rods of protein, called stereocilia, emerging from one end. When the BM moves at the location of a hair cell, the rods are deflected as if hinged at their bases. Such deflections in one direction increase the release of a chemical transmitter substance at the base (other end) of the cells, and deflections in the other direction inhibit the release. The variations in the concentration of the chemical transmitter substance act at the terminal ends of auditory neurons, which are immediately adjacent to the bases of the IHCs. Increases in chemical transmitter substance increase discharge activity in the nearby neurons, whereas decrements in the substance inhibit activity. Changes in neural activity thus reflect events at the BM. These changes are transmitted to the brain via the auditory nerve, the collection of all neurons that innervate the cochlea.
The steps described above are illustrated in the top panel of Fig. 100.1 , which shows a cartoon of the main anatomical structures, including the tympanic membrane, the three bones of the middle ear, the oval window, the BM, the IHCs, and the adjacent neurons of the auditory nerve.

Loss of Hearing
The principal cause of hearing loss is damage to or complete destruction of the sensory hair cells. Unfortunately, the hair cells are fragile structures and subject to a wide variety of insults, including but not limited to genetic defects, infectious diseases (e.g., rubella and meningitis), overexposure to loud sounds, certain drugs (e.g., kanamycin, streptomycin, and cisplatin), and aging. In the deaf or deafened cochlea, the hair cells are largely or completely absent, severing the connection between the peripheral and central auditory systems. The function of a cochlear prosthesis is to bypass the (missing) hair cells by stimulating directly the surviving neurons in the auditory nerve.
The anatomical situation faced by designers of CIs is illustrated in the bottom panel of Fig. 100.1 . The panel shows a complete absence of hair cells. In general, a small number of cells may remain for some patients, usually in the apical (low-frequency) part of the cochlea.
Without the normal stimulation provided by the hair cells, the peripheral parts of the neurons (between the cell bodies in the spiral ganglion and the terminals within the organ of Corti) undergo “retrograde degeneration” and eventually die ( ). Fortunately, the cell bodies are far more robust. At least some usually survive, even for prolonged deafness or virulent etiologies such as meningitis ( ).
Electrical Stimulation of the Auditory Nerve
Direct stimulation of the nerve is produced by currents delivered through electrodes placed in the scala tympani (ST), one of three fluid-filled chambers along the length of the cochlea. A cutaway drawing of the implanted cochlea is presented in Fig. 100.2 . The figure shows a partial insertion of an array of electrodes into the ST. The array can be inserted through an incision in the round window membrane, a drilled enlargement of the area ordinarily occupied by the membrane, or a drilled opening (“cochleostomy”) near the basal end of the cochlea and as illustrated in the figure.

Different electrodes in the array may stimulate different subpopulations of neurons. As described previously, neurons at different positions along the length of the cochlea respond to different frequencies of acoustic stimulation in normal hearing. Implant systems attempt to mimic or reproduce this “tonotopic” encoding by stimulating basally situated electrodes (first turn of the cochlea and lower part of the drawing) to indicate the presence of high-frequency sounds, and by stimulating electrodes at more apical positions (deeper into the ST and ascending along the first and second turns in the drawing) to indicate the presence of sounds with lower frequencies. Closely spaced pairs of bipolar electrodes are illustrated here, but arrays of single electrodes that are each referenced to a remote electrode outside the cochlea may also be used. This latter arrangement is called a “monopolar coupling configuration” and is used in all present-day implant systems that are widely applied worldwide; one such array is illustrated in Fig. 100.3 .

The spatial specificity of stimulation with an ST electrode most likely depends on a variety of factors, including the orientation and geometric arrangement of the electrodes, the proximity of the electrodes to the target neural structures, and the condition of the implanted cochlea in terms of nerve survival and ossification. An important goal of electrode design is to maximize the number of largely nonoverlapping populations of neurons that can be addressed with the electrode array. Present evidence suggests, however, that no more than four to eight sites are effective using current designs and placements of electrode arrays, even for arrays with as many as 22 electrodes (e.g., ). Most likely the number of effective sites is limited by substantial overlaps in the electric fields from adjacent (and more distant) electrodes. The overlaps are unavoidable for electrode placements in the ST, as the electrodes are “sitting” in the highly conductive fluid of the perilymph and additionally are relatively far away from the target neural tissue in the spiral ganglion. A closer apposition of the electrodes next to the inner wall of the ST would move them a bit closer to the target cells (see Fig. 100.2 ), and such placements have been shown in some cases to produce an improvement in the spatial specificity of stimulation ( ). However, a large gain in the number of independent sites may well require a fundamentally new type of electrode, a fundamentally different placement of electrodes, or optical rather than electrical stimulation (e.g., ). In addition, drug-induced growth of neural processes (“neurites”) from the ganglion cells toward the electrodes in the ST may well increase both the dynamic range and the spatial specificity of electrical stimulation by those electrodes (e.g., ).
Fig. 100.2 shows a complete presence of hair cells (in the labeled organ of Corti) and a pristine survival of cochlear neurons. However, the number of hair cells is zero or close to it in cases of complete hearing loss. In addition, survival of neural processes peripheral to the ganglion cells (the “dendrites”) is at least unusual in the totally deafened cochlea, as noted previously. Survival of the ganglion cells and central processes (the central axons) ranges from sparse to substantial. The pattern of survival is in general not uniform, with reduced or sharply reduced counts of cells in certain regions of the cochlea. In all, the neural substrate or target for a CI can be quite different from one patient to the next. A detailed review of these observations and issues is presented by .
Electrode arrays in current widespread use are thinner and more flexible than their predecessors. The array illustrated in Fig. 100.3 , for example, is quite thin and flexible and has “wavy” interconnecting wires that contribute to the flexibility ( ). These aspects of this particular design allow deep insertions into the ST and the possibility to address neurons along most of the length of the cochlea ( ).
Components of Cochlear Implant Systems
The components shared by all CI systems in current widespread use are illustrated in Fig. 100.4 and include a microphone for sensing sound in the environment; a processor to transform the microphone input into a set of stimuli for the implanted array of electrodes; a transcutaneous link for the transmission of power and stimulus information across the skin; an implanted receiver/stimulator to decode the information received from the radio-frequency signal produced by an external transmitting coil and then generate stimuli using the instructions obtained from the decoded information; a cable to connect the outputs of the receiver/stimulator to the electrodes; and the array of electrodes. These components must work together as a system to support excellent performance, and a weakness in a component can degrade performance significantly. For example, a limitation in the data bandwidth of the transcutaneous link can restrict the types and rates of stimuli that can be specified by the external processor, and this restriction can in turn limit performance.

One “component” that is not illustrated in Fig. 100.4 is the biological part central to the auditory nerve (the nerve is at the right and is colored yellow in the figure), including the auditory pathways in the brainstem and the auditory cortices of the implant recipient. As described in detail in , and , this part varies in its functional integrity and capabilities across patients, and is at least as important as the other parts in determining outcomes with implants.
Transformation of Input Sounds Into Stimuli for the Implant
An important aspect of the design for any type of sensory neural prosthesis is how to transform an input from a sensor or array of sensors into a set of stimuli that can be interpreted by the nervous system. The stimuli can be electrical or tactile, for example, and usually involve multiple sites of stimulation corresponding to the spatial mapping of inputs and representations of those inputs in the nervous system. One approach to the transformation (and probably the most effective approach) is to mimic or replicate at least to some extent the damaged or missing physiological functions that are bypassed or replaced by the prosthesis.
For CIs, this part of the design is called the processing strategy. As noted previously, advances in processing strategies have produced quite large improvements in the speech reception performance of implant patients, from recognition of a tiny percentage of monosyllabic words with the first strategies and multisite stimulation, for example, to recognition of a high percentage of the words with current strategies and multisite stimulation.
One of the simplest and most effective approaches for representing speech and other sounds with present-day CIs is illustrated in Fig. 100.5 . This is the continuous interleaved sampling (CIS) strategy ( ), which is a processing option in all implant systems now in widespread clinical use and the basis for other processing options in current use ( ).

The CIS strategy filters speech and other input sounds into bands of frequencies with a bank of bandpass filters. Envelope variations in the different bands are represented at corresponding electrodes in the cochlea with modulated trains of biphasic electrical pulses. The envelope signals extracted from the bandpass filters are compressed with a nonlinear mapping function prior to the modulation to map the wide dynamic range of sound in the environment (around 90 dB) into the narrow dynamic range of electrically evoked hearing (about 10 dB or somewhat higher). The output of each bandpass channel is directed to a single electrode, with low-to-high channels assigned to apical-to-basal electrodes, to mimic at least the order, if not the precise locations, of frequency mapping in the normal cochlea. The pulse trains for the different channels and corresponding electrodes are interleaved in time, so that the pulses across channels and electrodes are nonsimultaneous. This eliminates a principal component of electrode interaction, which otherwise would be produced by direct vector summation of the electric fields from different (simultaneously stimulated) electrodes. The corner or “cut-off” frequency of the low-pass filter in each envelope detector typically is set at 200 Hz or higher, so the fundamental frequencies of speech sounds are represented in the modulation waveforms. CIS gets its name from the continuous sampling of the (compressed) envelope signals by rapidly presented pulses that are interleaved across electrodes. Between 4 and 24 channels (and corresponding stimulus sites) have been used in CIS implementations to date.
Other strategies have also produced outstanding results, including the n -of- m ( ), spectral peak ( ), advanced combination encoder ( ), fine structure processing ( ), “HiResolution” ( ), HiResolution 120 ( ), CIS+ ( ), and “high-definition” CIS ( ) strategies. Detailed descriptions of these and prior strategies are presented in and . In addition, considerably more information about the CIS strategy is presented in , and .
Performance With the Present-Day Unilateral Implants
Each of the processing strategies in widespread use today allows mean word recognition scores in the order of 50%–55% correct and mean sentence recognition scores in the order of 70%–90% correct. Variability is high, with some listeners achieving scores of 90%–100% correct while others achieve scores of only 10%–20% correct.
Results for postlingually deafened adults and from two large studies conducted approximately 15 years apart are presented in Fig. 100.6 . The circles show scores for the individual subjects who were tested at different times after the initial fittings of their CIs, as indicated by the different columns in each of the panels. The data in the left two panels are a superset of those published in and include additional data at more test intervals for many of the subjects (additional data courtesy of Prof. Dr. Jan Helms and first published in ), and the data in the right two panels are from tests of all postlingually deafened adults who were implanted at the Vanderbilt University Medical Center (VUMC) from the beginning of 2011 through to early 2014 ( ; data courtesy of Dr. René Gifford). Some of the VUMC patients received implants on both sides, and for those patients each side was tested. In contrast, all persons participating in the Helms et al. study received an implant on one side only. In all, 55 ears were tested in the Helms et al. study and 267 ears were tested in the VUMC study.
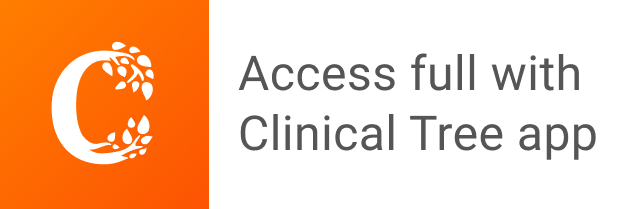