The Evolution of Neurons
Robert W. Meech
5.1 Introduction
In a letter to Charles Darwin dated July 20, 1875 George Romanes speculated about the evolutionary origins of the nervous system. He wrote:
My dear Mr. Darwin, …. physiologists have been so long accustomed to associate the phenomena of reflex action with some such distinguishable system, I was afraid that they might think me rather audacious in propounding the doctrine, that there is such a thing as reflex action without well‐defined structural channels for it to occur in. But if you have found something of the same sort in plants, of course I shall be very glad to have your authority to quote.
Reflexes might well be the basis of all behavior (Sechenov, 1863 [1965]) but Romanes worried that medusae appeared to exhibit them without the mediation of nerves.1
And I think it follows deductively from the general theory of evolution, that reflex action ought to be present before the lines in which it flows are sufficiently differentiated to become distinguishable as nerves.
Believing that he and Darwin had each discovered a proto‐nervous system, Romanes concluded that reflex function was not dependent on the prior evolution of neurons. Instead, reflex function appeared early in the course of evolution and then the neuron, the structure that enabled such functions to be coordinated efficiently, followed on.
Some 35 years later G. H. Parker (1911) proposed that nervous systems came into being in three successive stages. At the outset there were independent effectors such as the myocytes of the sponge osculum or the nematocytes of the cnidaria.2 Then receptor cells evolved from undifferentiated epithelia. Finally “central nervous organs would begin to differentiate in the region between receptors and effectors.” (Parker, 1911, p. 224) Initially they would be “organs of transmission whereby the whole musculature could be brought into coordinated action from a single point on its surface (Parker, 1911 pp. 224–225).” Later they would become “the storehouse for the nervous experience of the individual.” (Parker, 1911 p. 225) With this theory Parker (1910) consciously displaced an older idea, proposed by Oscar and Richard Hertwig (1878) that nerve and muscle cells had evolved simultaneously (see Figure 5.1).

Figure 5.1 Diagram to Illustrate the Way in which Undifferentiated Epithelial Cells Give Rise to the Components of a Reflex Arc according to (A) Kleinenberg (1872); (B) Hertwig and Hertwig (1878); and (C) Parker (1910).
(A) a single cell in an epithelial layer (top) differentiates to form a neuromuscular cell which then separates to give a neuron and a muscle cell. (B) three separate epithelial cells differentiate to form a muscle cell, a nerve cell, and a ganglion cell. (C) one epithelial cell differentiates to form an independent effector; subsequently a second epithelial cell differentiates to form a nerve cell. Parker (1910) finds no evidence for Kleinenberg’s neuromuscular cells and opposes the Hertwigs’ hypothesis of simultaneous differentiation of nerve and muscle, because of evidence in sponges of contractile elements without nerves. Whether the sponge’s immediate ancestor might have already evolved nerves is a matter of current debate (see Ryan & Chiodin, 2015).
Adapted from Parker, 1911.
Carl Pantin (1956, p. 173) favored the Hertwig’s earlier idea on the grounds that “The Metazoan behavior machine did not evolve cell by cell and reflex by reflex. From its origin it must have involved the structure of the whole animal.” Pantin (1956) and Passano (1965) followed Sherrington in believing that integration was an essential property of any nervous system, the first appearance of which would be as a network of neurons because a simple three‐component reflex would be incapable of producing an integrated output.
In the 1960s it became generally recognized that animals did more than simply respond to sensory input. Even the simplest nervous systems could generate activity internally. One proposal was that assemblages of electrically coupled “protomyocytes” might develop unstable “pacemaker” regions, becoming specialized for activity initiation with “The specialization of the nerve cell for the conduction … seen as a secondary development” (Passano, 1965, p. 308). Based on his studies on the cnidaria, Passano suggested that this secondary development might arise from regions of the neuronal nerve net that had become specialized for “through‐conduction,” rather in the way a bypass might be installed once traffic through the city center becomes too congested.
Another important development in the 1960s was the discovery of epithelial conduction (Mackie, 1964, 1965; Mackie & Passano, 1968; Roberts, 1969) and the possibility that neuronal conduction evolved from excitable epithelia as a means to give precision to a system that would otherwise generate only generalized responses to excitation (Horridge, 1968; Mackie, 1970). Other proposals at about that time included the idea that impulse conduction was secondary to the delivery of chemical agents to distant sites (Lentz, 1968). Such chemicals might perhaps include trophic agents delivered by what we now call axonal transport.
Carl Pantin, while emphasizing the complexity of “the neuro‐muscular machine” that executes behavior in higher animals, recommended studying its origin and evolution and encouraged the reexamination of “the capabilities of simple systems in the light of contemporary physiological knowledge” (Pantin, 1965, pp. 171, 178). To begin the process we need to define exactly what a neuron is, but this turns out to be a surprisingly difficult task. The ideas of Romanes, the Hertwigs, Pantin, and Passano, as summarized above, suggest that such a definition should encompass:
- the early stages of evolution when the functions of the nervous system might be performed by non‐neuronal structures (Romanes, 1875).
- the connection of independent effectors with receptor cells (Parker, 1919).
- the difficulty of making a simple three component reflex produce an integrated output (Pantin, 1956).
- the ability to initiate repetitive activity (Passano, 1965).
A definition that fully satisfies all criteria may well be impossible but as a start: “A neuron is a constituent of the nervous system; its function is to communicate with other cells by way of electrical and/or chemical signals; it is a form of communication that usually takes place via synapses.” The word “communication” bears examination: It creates the impression that neurons simply relay what Sherrington (1906) called “states of excitement.” However, as living systems became more complex, and their sense of the environment expanded, it became essential to integrate the different streams of information, or there would be no guarantee of an appropriate response. The word “communication” thus carries a lot of baggage—it needs to convey a capacity for carrying highly processed information.
The aim of this chapter is to consider the evolution of both aspects of neuronal function: signal transmission and integration of information. It begins with an account of the Porifera and the question of whether the sponges contain the elements of a proto‐nervous system. This is followed by a discussion of whether neurons may have evolved more than once. The publication of the genomes of the ctenophores Mnemiopsis leidyi (Ryan et al., 2013) and Pleurobrachia bachei (Moroz et al., 2014) lends some support to this possibility as it appears that neurons may have evolved independently in the Ctenophora and the Cnidaria. Either this, or “much of the genetic machinery necessary for a nervous system was present in the ancestor of all extant animals” (Ryan et al, 2013, p. 1342)3 being secondarily lost in the lineage that lead to the sponges. Much of the rest of the review is taken up with a summary of the properties of the cnidarian nervous system, because it is here that we have the most clues to nervous system origins.
5.2 Non‐neuronal Reflexes in Porifera
According to Robert Grant (1936, p. 108) sponges lack “perceptible nervous or muscular filaments or organs of sense.” The key word here is “perceptible”: Only after many years of careful histological study was it finally established that sponges really do not possess nerve‐like elements (Jones, 1962; Pavans De Ceccatty, 1974), which is not to say that they do not exhibit reflexes or conduct electrical impulses and show evidence of contractile apparatus (see Leys & Meech, 2006 for references).
Almost all sponges are filter‐feeders, the water being pumped by beating flagella through a system of delicate canals. The danger is that these canals are susceptible to damage by sediment in the incoming water, a problem that different classes of sponge counter in different ways. The cellular sponges (Calcarea and Demospongiae) regulate the flow by compressing the flagellated chambers or by contracting the canal system (see Leys & Meech, 2006), while the glass sponge Rhabdocalyptus dawsoni (Hexactinellida) provides an entirely different model based on an electrical mechanism.
Hexactinellids are not the easiest animals to record from, but they do recognize, and accept, grafted cells. It is from these grafts that all‐or‐nothing Ca2+‐based action potentials (APs) may be recorded in response to electrical stimulation (Leys & Mackie, 1997; Leys, Mackie, & Meech 1999). These APs, which last at least 5 seconds and propagate at a speed of 0.17–0.3 cm/s, are associated with arrested water pumping (see Figure 5.2). Much of the soft tissue in Rhabdocalyptus consists of a single syncytium, which means that impulses can propagate freely. Other sorts of cells are attached to the reticulum by “plugged junctions.” As these junctions are little more than protein‐filled holes, impulses can pass through them too and arrest the beating of flagellated cells. Presumably the entry of Ca2+ during the impulse affects the ciliary beating in much the same way that it does in Paramecium (Naitoh & Eckert, 1969) and that is what brings water pumping to a halt.

Figure 5.2 Impulse Generation in the Glass Sponge Rhabdocalyptus dawsoni.
(A) Dispersed and reaggregated sponge cells (g) 24 hours after having been placed on the surface of a piece of the “donor” sponge. The two parts of the graft are connected by “toffee”‐like strands (arrows) that establish syncytial continuity between graft and sponge. (B) Conduction time: an impulse initiated at (S) propagates past recording electrodes (R1, R2). Stimulating and recording electrodes arranged in a line as shown; the calculated conduction velocity was about 0.3 cm s−1. An asterisk marks the stimulus artifact.
From Leys, Mackie, & Meech, 1999.
It is evident that complex defensive behavior, precisely coordinated if somewhat slow, can arise without the participation of neurons. The freshwater sponge Ephydatia muelleri provides another example. It protects itself from damaging sediment by a non‐neural reflexive “sneeze” (Elliott & Leys, 2007). The sponge first inflates; there is a period of hiatus, and then an explosive contractile spasm causes accumulated indigestible matter to be expelled through the osculum. Observation of this stereotyped inflation–contraction behavior was made possible by the transparency of the preparation; the authors were able to watch cells crawling through the sponge interior and note that they arrested their movement for as much as 10 minutes while the wave of contraction passed by, as if the spasm was coordinated by a diffusible chemical messenger (Elliott & Leys, 2007). The propagated contractions can be mimicked using low levels of glutamate and prevented with the metabotropic glutamate receptor antagonist, L‐2‐amino‐3‐phosphonopropionate (L‐AP3) or the competitive inhibitor kynurenic acid (Elliott & Leys, 2010).
Sponge larvae exhibit another form of behavior coordinated without nerves. According to Nielsen (2008, p. 254), humans, as well as all other eumetazoans, are “descendents of a derived sponge larva.” Most sponge larvae are covered by a ciliated columnar epithelium (Maldonado & Bergquist, 2002) whose beat provides the force for forward movement. The larvae of Reniera are negatively phototactic in the first few hours of life (Leys & Degnan, 2001). In addition to the short cilia already mentioned, larvae have a “tuft” of pigmented epithelial cells, each of which gives rise to a single long cilium (Maldonado, 2006). These long cilia, which are assumed to act as a kind of rudder, take up “relaxed,” “contracted” or “expanded” arrangements depending upon whether the light intensity is increased or decreased. How light brings about the transformation is not known but the end result is that a sensory input is integrated with directed movement, enabling Reniera larvae to settle in shaded regions of a reef.
Whatever their position on the phylogenetic tree, sponges make a good, if somewhat static, living in the absence of a nervous system. Even if the sponge’s immediate ancestor had the genetic machinery to build a nervous system, the evolution of a rigid skeleton in the presence of a relatively stable environment would make rapid response times unnecessary; diffusion‐based chemical coordination would be quite sufficient. Consequently, the slow electrical conduction seen in Rhabdocalyptus should not be thought of as a degraded version of neural conduction but as an example of how easy it is for natural selection to assemble the molecular architecture needed for propagating signals.
5.3 The Ctenophore Enigma
Ctenophores are voracious predators whose locomotion depends on the beating of (usually) eight bands of cilia, an arrangement that makes them highly maneuverable (Tamm, 2014). They can hunt their prey by smell (“chemokinetically”) (Swanberg, 1974) and can escape from predators (Kreps, Purcell, & Heidelberg, 1997). Feeding responses are triggered when prey contacts either the lips (Beroe) or the tentacles (Pleurobrachia). Their orientation is influenced by information from a statocyst in the aboral organ.
It is unfortunate that we know so little about the ctenophore nervous system and its electrophysiology. What little we do know has had to be inferred from anatomical and behavioral studies. The ctenophore nervous system takes the form of two distinctly separate nerve nets (Jager et al., 2011) with a high density of nerve elements at the aboral sensory pole and along the ciliary bands; bipolar and tripolar neurons are generally distributed under and between the ectodermal epithelial cells (Hernandez‐Nicaise, 1973). In Beroe the nerve net is denser around the lips and in Pleurobrachia and Hormiphora two thick strands of fibers and neurons connect the aboral organ and the tentacles. There are frequent and highly differentiated synaptic contacts suggestive of chemical transmission and electrical events resembling excitatory synaptic potentials have been recorded from isolated muscle fibers (see Meech, 2015). To judge from the density of nerve elements any integration of the animal’s responsive movements must take place either at the ciliary bands, which are subject to a variety of sensory inputs relating to prey capture and balance, or at individual muscle fibers in the body wall.
Unlike the cnidaria (see later), which have a myoepithelium, ctenophores have “true” muscle cells each with its own neuronal input. In Beroe ovata, muscle cells are of three main types, longitudinal, radial, and circular (Hernandez‐Nicaise & Amsellem, 1980), each fiber running freely within the transparent extracellular matrix, the mesogloea. Longitudinal fibers may be many centimeters long in large specimens because some travel the whole length of the body; radial fibers are often as much as 40 µm in diameter. These multinucleate cells resemble vertebrate smooth muscles in that they lack striations or a transverse system of T‐tubules (Hernandez‐Nicaise & Nicaise, 1986) and they contract upon stretching. Their sarcoplasmic reticulum makes up less than 1% of the myofilament volume (Cario, Malaval, & Hernandez‐Nicaise, 1995), contraction depending on influx of Ca2+ from the bathing medium rather than intracellular Ca2+ release. The characteristics of the ion channels in the membrane of each of the different classes of muscle establish the ion currents that mold the form of the muscle AP. This sets the amount of calcium entering the cell and therefore contributes significantly to its contractile properties (Hernandez‐Nicaise, Mackie, & Meech, 1980; Bilbaut, Meech, & Hernandez‐Nicaise, 1988; Bilbaut, Hernandez‐Nicaise, Leech, & Meech, 1988).
Radial fibers are capable of generating a train of APs in response to a maintained depolarization, whereas excitation of longitudinal muscle elicits only a single plateau‐shaped AP and a short‐lived contractile response such as might open the mouth during swallowing. It is the maintained tension generated by the radial fibers as they contract against the viscoelastic mesogloea that keeps the animal stiff, and this suggests that the nerve net must provide continuous excitation. Whether ctenophore neurons do any more than simply distribute excitation remains a key question.
5.4 The Cnidarian Nervous System
“I think everyone will feel it to be obviously true that if ganglionic action is ever to receive any considerable elucidation, the medusae are by far the most promising structure to yield it.”
(Romanes, 1880)
Textbooks have, for far too long, given the impression that nervous systems evolved in a simple progression from nerves to nerve nets, from nerve nets to ganglia, and from simple ganglia to the complexities of the human brain, with each stage awaiting dramatic changes in the host species’ genome. In fact, a more likely scenario is that early metazoans contained collections of genes that afforded nervous system construction—not in the early stages as a necessity, but certainly as a possibility—with the form of the first nervous systems depending as much on the properties of their host’s life cycle, development, and skeleton as on limitations imposed by their neuronal components.
Two examples will illustrate the links between body plan, life cycle, and neuronal function. According to Passano & Pantin (1955), the form of the sea anemone Calliactis parasitica resembles a giant sense organ. The whole body is structured like an integrative surface with a thin tentacular disc sitting ear‐drum‐like on a stout cylindrical column. Sensory information is gathered with maximum sensitivity from all over the anemone’s surface. Most sea anemones have a sedentary existence and, as we shall see, neurons in the form of a diffuse nerve net are well‐adapted to connect the sensory input, in the form of vibrations, to the necessary motor output.
In contrast, Cnidaria that go through a medusoid stage have evolved the more condensed nervous systems that permit the rapid, complex behavior necessary for survival as a free‐living entity. Like other ambush foragers (Colin, Costello, & Klos, 2003; Dabiri, Colin, Katija, & Costello, 2010), the hydrozoan Aglantha digitale (Figure 5.3A) lives by “sink‐fishing” spending part of its time sinking with its tentacles fully extended trapping prey, and part of its time regaining height in the water column by performing a series of slow swims. During the swimming phase of the cycle it rights itself with a series of asymmetrical contractions, and then swims upward with its tentacles contracted (Mackie, 1980). As shown in Figure 5.3C Aglantha can also generate fast swims to escape from predators (Donaldson, Mackie, & Roberts, 1980). This varied behavior emanates from a highly organized nervous system. Aglantha has nerve tracts that run from the tentacle‐fringed margin to control its lips during feeding; has pacemaker neurons responsible for rhythmic swimming; has inhibitory synapses to coordinate swimming and feeding; and has giant motor axons that support its rapid escape (Mackie & Meech, 1985; Mackie, Marx, & Meech, 2003; Meech & Mackie, 1995; Roberts & Mackie, 1980). Furthermore, its electrically coupled myoepithelium, which is organized into “fields” by a grid of lateral neurons (Figures 5.3B, 5.4C; Kerfoot, Mackie, Meech, Roberts, & Singla, 1985), is capable of graded and regionalized contractions.

Figure 5.3 Swimming in Aglantha digitale.
(A) Transverse section through Aglantha digitale (line drawing from a photograph by Claudia Mills). The animal is resting at the end of a swimming cycle. Many fine tentacles extend from the margin at the base of the bell. The margin also contains the neurons responsible for generating slow swimming. (B) Diagram showing two motor giant axons in synaptic contact with the myoepithelium and electrically coupled to an array of lateral motor neurons. The radial canal (shaded area) runs in the endoderm below. Intracellular recording sites R1, R2, and R3 are shown. From Kerfoot et al., 1985. Inset (below right): overshooting action potential recorded at site R2 with the corresponding electrical event in the nearby myoepithelium, R3. Axon resting potential, −62 mV; myoepithelium resting potential, −77 mV. Inset (below left): low‐amplitude slow swim spike recorded at sites R1 and R2 in response to a brief depolarizing current pulse (*); a small increase in the intensity of the injected current was sufficient to elicit an overshooting action potential in the same axon; axon resting potential, −72 mV. Mackie and Meech, 1985. (C) Video frames of an escape swim elicited by a glass probe used to disturb vibration receptors at the base of the tentacles; time (ms) after stimulus shown at the bottom of each frame. Frames were captured at 1/300 second. During an escape swim the jellyfish bell contracts uniformly along its length but during recovery the base recovers before the mid‐bell. Meech, 2015.
The way the nervous system is organized in each of the two main cnidarian body patterns broadly defines their behavioral capabilities. In the usually sedentary anthozoan polyp, the nervous system takes the form of a diffusively conducting nerve net, although some condensation is seen in some sea anemones and corals that exhibit quite complex, apparently coordinated, movements. Condensation is more obvious in the medusa of the Scyphozoa, Cubozoa and Hydrozoa, and these more accessible modular nervous systems, have revealed more of their integrative mechanisms. In this review I shall focus, almost exclusively, on examples from the Hydrozoa with only a short summary to show some differences in the Scypho‐ and Cubozoa.
5.4.1 Complex Behavior and the Anthozoan Nerve Net
When Carl Pantin started his classic sea anemone experiments it was believed that a nerve net would conduct excitation in all directions according to the strength of the stimulus; in other words, the greater the strength of the stimulus, the farther its effect propagated. The implication was that the electrical signal died away as it traveled, rather than being the all‐or‐nothing phenomenon that Adrian (1912) had demonstrated in frog nerves.
A visit to Naples marine station gave Pantin (1935a, 1935b, 1935c) the opportunity to correct this idea. Following a series of electrophysiological experiments on crab nerves he decided to compare them with the closure reflex of the sea anemone Calliactus parasitica. He soon realized that the size and nature of the reflex was completely independent of the strength of individual stimuli. In fact, it was controlled simply by the frequency and number of shocks, just like the “all‐or‐nothing” responses of vertebrate axons. The secret of Pantin’s success was that he used physiological stimuli; previous workers had used either individual shocks or high‐frequency shocks that ignored the refractory period. A striking feature of the sea anemone nervous system was its very low background activity; in a normal resting state an impulse was recorded less than once in every 10 seconds. Even when artificially stimulated, a rate of one AP/second evoked a maximal response. “The whole scheme is thus 100 to 1000 times slower than that which characterizes the nervous organization of the vertebrates.” (Pantin, 1935b, p. 154)
Calliactus has a very strong defensive withdrawal response which can be elicited using either mechanical or electrical stimuli. The main muscles involved are the ectodermal longitudinals in the tentacles and the endodermal marginal sphincter, which is at the top of the column and closes it off like a draw‐string bag (Hall & Pantin, 1937; Pantin, 1935a). Pantin found that although a single stimulus produced no response at all, multiple stimuli produced a graded response, the amplitude and extent of which depended on frequency. Having already realized that cnidarian nerves were all‐or‐nothing systems Pantin concluded that although a single impulse might not cause contraction by itself, it could facilitate transmission. “The nerve net consists of units that behave like true nerve. It is characteristic that it tends to conduct stimuli in all directions; but its most striking feature is the extreme degree to which facilitation is developed both within the net and between the net and the muscles.” (Pantin, 1935a, p. 136) Facilitation between the net and the muscles would explain why two or more stimuli were needed to initiate contraction, while facilitation within the net would mean that the contraction would spread out with increasing numbers of stimuli.
There is a striking absence of information about the mechanism of this facilitation in either the nerve net or at the neuromuscular junction. It is possible that it is a presynaptic phenomenon but this is far from certain. A form of neuromuscular facilitation studied in the dyphid siphonophore Chelophyes arises from the progressive inactivation of a repolarising K+ current during a series of muscle APs (Inoue, Tsutsui, & Bone, 2005), and a similar inactivating current has been recorded from isolated Calliactis muscle cells (Holman & Anderson, 1991). Muscle APs recorded by Holman and Anderson (1991) get progressively longer during a train as expected from a decline in the availability of repolarizing K+ current. Since the strength of contraction depends upon the amount of Ca2+ influx the longer the AP the stronger the contraction will be. If this mechanism provides the basis for facilitation in Calliactis, it should fade as the muscle K+ channels recover from inactivation, but no data is available for this characteristic at present.
Although superficially similar in form, different species of sea anemone have evolved markedly different ways of carrying out defensive withdrawal. In Metridium senile (plumose anemone) the major muscles involved are the longitudinal retractors of the mesenteries. These muscles contract and withdraw the oral disk and it is only after this withdrawal is completed that the sphincter contracts and provides a cover. In another species, Anemonia viridis (snakelocks anemone), Pantin could find no reaction that was obviously protective; the sphincter is barely developed in this species and the disk does not close (Pantin, 1935b). Stimuli that might produce withdrawal in Calliactis or Metridium appear to evoke a feeding response in Anemonia. There is clearly scope for exploring the links between the nature of the environmental stresses, the mechanism of defense and the molecular basis for facilitation in each of these different species.
5.4.2 Mechanisms of Neuronal Integration in Medusae
Most jellyfish are radially symmetrical with either a bell or umbrella shape (see Figures 5.3A and 5.5A for bell‐shaped hydrozoan jellyfish). The body wall consists of two layers of epithelium separated by an elastic jelly‐like layer, the mesogloea (Figure 5.4A). The outer (exumbrella) epithelium is a layer of simple epithelial cells. The inner (subumbrella) epithelium is made up of a sheet of myoepithelial cells each of which consists of an elongated muscle with a cell body connected to it by a short neck (Figure 5.4B). The bell, or umbrella, is fringed with tentacles and hanging down within the subumbrella cavity is the peduncle with the mouth, or manubrium, at its end. The mouth leads to the radial canals which travel up the peduncle towards the bell apex and then on down the subumbrella to the rim or margin. The radial canals are lined with beating cilia, which distribute the products of digestion around the body. They divide up the subumbrellar myoepithelium into sections and are continuous with the ring‐shaped lateral canal, which travels all around the margin of the bell. Also at the margin, on either side of the mesogloea, are the inner and outer nerve rings.

Figure 5.4 Hydrozoan Anatomy.
(A) Per radial section to show the marginal nerves. Running in the nerve rings at the base of the velum are about 800 neurones; most are less than 1 µm in diameter, but the motor giant, ring giant, and tentacle giant axons are significantly larger. The nerves that cross the mesogloea at the base of the velum connect the nerve rings. Hair cells make contact with the ring giant axon directly. Connections passing around the sides of the tentacle connecting the tentacle giant axon to the outer nerve ring have been omitted. Also omitted is the small nerve bundle that runs beside the motor giant. Mackie & Meech, 1995a. (B) Diagram to show the position of a giant motor axon within the myoepithelium between the muscles and their cell bodies. An isolated myoepithelial cell is shown at the bottom of the figure to clarify its structure. The muscles run in a circular direction. Lateral motor neurons, which also run below the cell bodies, make electrical contact with the giant axon and innervate the myoepithelium in distinct fields. The small axon bundle runs parallel to the giant axon. The radial canal which runs within the endoderm, parallel to the giant axon is not shown.
Adapted from Kerfoot 1985.
(C) Schematic layout of nerves and muscles in the subumbrella of Neoturris breviconis to show their relationship to the radial canal. Note the presence of circular smooth muscle.
Adapted from Mackie & Meech, 2008.
From a simple bell‐shaped structure the long course of natural selection has crafted a wide range of morphological variants. Each body form specializes in a particular foraging strategy and associated with these strikingly different patterns of behavior are variations in the neuronal ground plan. Some jellyfish, Aequorea victoria for example, are more umbrella‐shaped (oblate), the polar axis being shorter than the diameter. Others, such as Polyorchis and Aglantha are taller than they are wide. Aequorea forages/swims for more than 80% of the time (Colin & Costello, 2002; Colin et al., 2003) using a rowing motion to set up a steady feeding current that draws prey into the entrapping fringe of tentacles; Polyorchis and Aglantha engage in “sink‐fishing,” a type of foraging that uses intermittent jet‐propelled swimming for which their more streamlined prolate form is well suited.
The advantage to the swimming exhibited by oblate jellyfish is that it can be maintained for long periods. The disadvantage is that the trapped prey is so far removed from the mouth that, for transfer from the tentacles to take place, swimming must cease and the rim of the bell be drawn inwards. In Aequorea this is achieved by the contraction of discrete bands of radial muscles that run down the subumbrella from the top of the bell to the margin (Satterlie, 1985). Naturally, “sink‐fishing” has its advantages too. One is that during the non‐swimming phase jellyfish such as Aglantha deploy an extended field of tentacles to trap their prey. The tentacles are lined with beating cilia that set up water currents to draw the prey inwards (Mackie, Nielsen, & Singla, 1989). A disadvantage is that without swimming Aglantha will sink continuously in the water column. To swim upward again, it must shorten its widespread field of tentacles so as to reduce the effects of drag (Mackie, 1980).
Before examining the molecular basis of some of these behaviors in more detail, some account is necessary of the subumbrellar musculature and its neuronal innervation. Despite a relatively simple ground plan (or perhaps because of it) there are a large number of variations. In Neoturris breviconis (Figure 5.4C) the entire subumbrellar surface is covered by both circular striated muscle and radial smooth muscle while in other species, such as Stomatoca atra, the radial smooth muscle is confined to a thickened band above each of the radial canals. In Aglantha, radial muscle is completely absent from the subumbrella although it remains in the manubrium and the velum.
The radial muscles that play such an important role in Aequorea in bringing trapped prey to the manubrial lips also have an important defensive role. Many hydromedusae respond to agitation by exhibiting a behavior described by Hyman (1940) as “crumpling.” The response, which can be elicited by mechanical or electrical stimulation of the exumbrella, involves the contraction of the radial muscles of the bell and the longitudinal muscles of the tentacles so that the margin and tentacles are drawn up into the subumbrellar cavity. Crumpling is absent in Aglantha, which does not have radial muscle in its bell and which exhibits another form of defense: escape swimming (see Figure 5.3C; Donaldson et al., 1980).
In Polyorchis, the organisation of the swim motor neuron network, which extends in an arch at the apex of each subumbrellar quadrant (Lin, Gallin, & Spencer, 2001), suggests that excitation of the electrically coupled muscle sheet spreads from all four sides. The synchronous firing of the swim motor neurons around the nerve ring ensures that all quadrants contract together, producing symmetrical swimming movements. In Aglantha the motor axons travel up the bell next to each of the (in this case) eight radial canals. Symmetrical contraction during escape swimming is accomplished by an unusual ring‐shaped neuron, the ring giant, located in the outer nerve ring. This provides an almost coincident input into the eight giant motor axons. Excitation then spreads into the myoepithelium from neuromuscular junctions distributed along each axon. Electrical coupling between myoepithelial cells ensures that the depolarizing current spreads around the bell, but the spread of excitation is also promoted by lateral neurons (see Figure 5.3B) that are electrically coupled to the motor axons and innervate the myoepithelium in discrete, mostly non‐overlapping, fields (Kerfoot et al., 1985; Weber, Singla, & Kerfoot, 1982). As a consequence depolarizing junction potentials may be recorded at all sites in the myoepithelium.
In Aequorea, swimming movements are somewhat less symmetrical than in Aglantha. The myoepithelium is divided by 50 or more radial canals, and excitation is largely confined to individual stimulated segments. Swims are initiated by a burst of APs in the equivalent of the swim system in the inner nerve ring. In the subumbrellar myoepithelium the AP burst is translated into a flurry of junction potentials giving rise to a single long‐lasting AP (Satterlie, 2008). As with Aglantha there are junction potentials at all sites, and the widespread presence of neurites suggests that the spread of excitation within the myoepithelium depends on a subumbrellar nerve net. Local inhibition of this nerve net can prevent swimming contractions in restricted regions of the bell (Satterlie, 2008).
Although we might question speculations about neuronal evolution based on present‐day morphologies, it seems not unreasonable to conclude that the first medusa was broadly bell‐shaped and used its myoepithelium to swim and its tentacles to trap food. That being so, the rich variety summarized in this section gives us the means to explore the relationship between body form, behavior and neuromuscular function, so as to identify recurring mechanisms of integration that may have arisen in the earliest nervous systems. The main areas of interest are: (1) swimming, (2) control of tentacle length, (3) defense, (4) swimming inhibition and mouth manipulation during feeding.
5.4.2.1 Swimming.
Among the Hydrozoa one of the best worked out neural systems is in the anthomedusa Polyorchis penicillatus (Figure 5.5A) where the swim pacemakers are sufficiently large for intracellular recording (Spencer, 1981) and voltage‐clamp (Przysiezniak & Spencer, 1989). The trachymedusa Aglantha digitale, with its 40 µm diameter motor axons, has also been extensively studied under voltage and patch‐clamp (Meech & Mackie, 1993a, 1993b). A major difference between the two animals is that in Aglantha the pacemaker neurons and the swim motor neurons are separate entities whereas in Polyorchis the pacemaker neurons directly innervate the myoepithelium.

Figure 5.5 Modular Nature of Polyorchis penicillatus Nervous System.
(A) Line drawing of a Polyorchis specimen at rest. (B) Partial representation of the ring‐shaped neuronal networks showing known synaptic contacts. The muscle epithelium (blue) is directly excited by the swim motor neurons (red) in the inner nerve ring. They in turn receive an excitatory synaptic input from neurons of the “B” system (yellow) in the outer nerve ring and from unicellular receptors (orange). Excitatory inputs to the “B” system are shown arising in the ocelli (purple). Also shown is an excitatory pathway from the swim motor neurons to the “O” system (green) in the outer nerve ring; other connections may be present. Excitatory synapses are indicated by filled triangles (▾). All three systems consist of a ring of electrically coupled nerve cells.
Adapted from Spencer & Arkett 1984.
(C) Inactivating K+ currents recorded under voltage clamp in response to test commands to +50 mV. Each test command preceded by a conditioning command lasting 1 s. The superimposed current traces show the effect of the conditioning level (range −90 to −20 mV; 10 mV steps) on the availability of the inactivating current. At −20 mV the inactivating component (IKfast) is absent, leaving IKslow.
Adapted from Przysiezniak & Spencer, 1994.
5.4.2.1.1 Control by the nerve ring of hydrozoan jellyfish.
The pacemaker activity that provides for rhythmic swimming in hydrozoan jellyfish arises from nerve rings at the base of the bell. In Polyorchis (Figure 5.5A) the pacemaker is affected by ambient light levels and includes as many as three interconnected modules, each consisting of an electrically coupled ring of neurons (Spencer & Arkett, 1984). As indicated in Figure 5.5B, activity in the ‘swim system” is modified by inputs from the “B system” and the “O system.” In other jellyfish, such as Aglantha, the pacemaker system is unaffected by light.
5.4.2.1.2 The “swim system.”
When Polyorchis swim, motor neurons show slow, apparently endogenous, baseline oscillations even when high levels of Mg2+ are used to block synaptic and muscular activity (Satterlie & Spencer, 1983). They are electrically coupled via gap junctions (Anderson & Mackie, 1977; Spencer, 1975) and, as in other examples of electrically coupled neurons, would be expected to respond only to inputs that affect many cells in the network simultaneously (Willows & Hoyle, 1969). In fact the inputs need not be exactly coincident because their EPSPs take about 750 ms to reach a maximum and have an overall duration of 1.5 second (Spencer & Arkett, 1984; Mackie, Meech, & Spencer, 2012). This slow time course is an inevitable consequence of the electrical coupling and the low‐pass filtering arising from the series resistance and capacitance to ground of the postsynaptic cell membrane (Bennett, 1966; Bennett & Zukin, 2004; Spencer, 1981).
Na+, Ca2+ and K+ channels have been studied in dissociated swim system neurons under whole cell voltage‐clamp (Przysiazniak & Spencer, 1994). The outward K+ current (IKfast), which flows only transiently because it undergoes rapid inactivation, resembles a current thought to dominate the latter stages of the inter‐spike interval in repetitively firing neurons (Connor & Stevens, 1971). In Polyorchis, IKfast is half‐inactivated with the membrane set to −52 mV (Przysiazniak & Spencer, 1994) although the inward Ca2+ and Na+
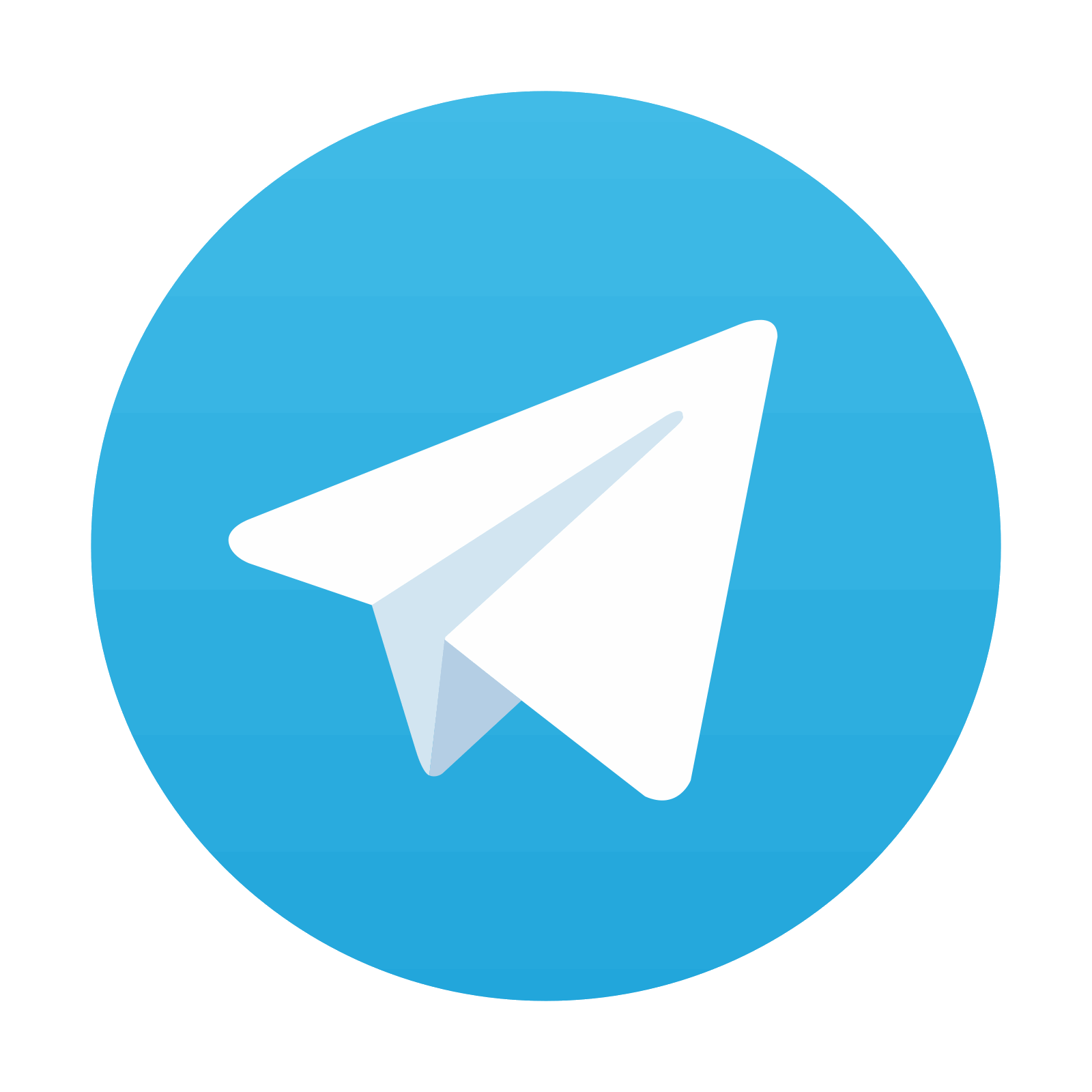
Stay updated, free articles. Join our Telegram channel
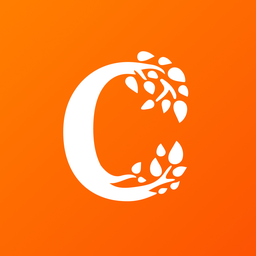
Full access? Get Clinical Tree
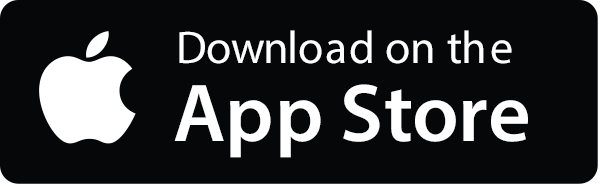
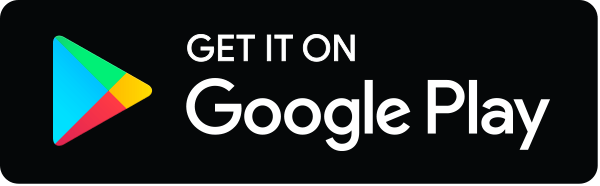