The First Nervous System
Nadia Riebli and Heinrich Reichert
6.1 Introduction
The origin of the first nervous system is an intriguing enigma. Stated in its simplest form, a nervous system can be defined as a set of interconnected neural cells that process information via electrical and/or chemical signals. In consequence, by definition, the first nervous system evolved after the evolutionary transition from unicellular to multicellular life forms. Since nervous systems allow integration of sensory input and coordination of motor output in a behaviorally relevant manner, there are obviously significant selective advantages in evolving more sophisticated and complex nervous systems. In animal evolution this has led to the emergence of centralized nervous systems which comprise distinct agglomerations of functionally specialized neurons, that may be subdivided into separate parts (ganglia), are interconnected by axon tracts (neuropil) and connect to the periphery via nerves (Arendt, Denes, Jékely, & Tessmar‐Raible, 2008). Moreover, in most extant bilaterian animals, nervous system centralization combined with cephalization has resulted in the appearance of brains, which are prominent anterior ganglia that receive major input from sense organs located on the head and send descending motor output to the somatic effector apparatus in the remaining body via nerve cords. In this review, we focus on the evolution of complex nervous systems from simple neural origins and consider evidence from comparative, developmental, and molecular genetic studies that shed light on this fascinating evolutionary process.
6.2 The Ambiguity of Nervous System Origins
A phylogenetic assessment of the origin of nervous systems based on currently available paleontological data is both enlightening and disappointing. On one hand, there is clear fossil evidence for the existence of complex nervous systems, including brains, in bilaterian animals that date back to at least 530–540 MYA (million years ago). Thus, the fossil records for ancestral arthropods and agnathan‐like vertebrates indicates that both groups already had brains and central nervous systems with features typical of extant arthropods, which are members of the protostome supergroup, and of extant vertebrates, which are members of the deuterostome supergroup (Fortey, 2000; Holland & Chen, 2001; Ma, Hou, Edgecombe, & Strausfeld, 2012; Northcutt, 2012; Tanaka, Hou, Ma, Edgecombe, & Strausfeld, 2013). This implies that centralized nervous systems with brains evolved before the protostome–deuterostome split in the urbilaterian ancestor of both major bilaterian supergroups. Centralization of nervous systems must have occurred earlier, probably after the split between bilaterians and radiate animals such as cnidarians which is dated at 600–630 MYA (Peterson et al., 2004). However, fossil evidence for nervous systems from this Precambrian period is scarce and difficult to interpret. Hence, although evidence for the existence of central nervous systems in the early Cambrian is solid, we are left with little information on the origin of the first nervous system from paleontology.
A phylogenetic evaluation of the origin of nervous systems based on comparative neuroanatomical analyses of extant animals is also ambiguous, albeit for different reasons.
First, the nervous systems of all extant animals are by definition modern in that they have had the same amount of time to evolve (hundreds of millions of years). Furthermore, this evolutionary process can lead to both increase and reduction of nervous system complexity. Thus, even when a nervous system appears to be rather simple and “primitive” in neuroanatomical respects, this simple morphology can be due to a secondary loss of more complex structures due to the environmental features that the animal has adjusted to and due to the requirements of its ecological niche. Hence, it is a priori unclear which, if any, of the living animals have nervous systems that reflect the original, primitive nervous system in the Precambrian ancestor of bilaterian and radiate animals.
Second, our understanding of animal phylogeny (see Figure 6.1) is currently in flux—and a source of considerable controversy—mainly due to the interpretation of new data from genetic and genomic analysis. As a result, it is often unclear which group of extant animals is basal and, thus, most likely to have a “primitive” type of nervous system. This is exemplified by the recent dramatic changes in “flatworm” phylogeny and their implications for brain evolution in bilaterians. Flatworms are classically considered to represent the simplest organizational form of all living bilaterians with a true central nervous system and, based on their simple body plans, have been traditionally grouped together in a single phylum at the base of the bilaterians (Bullock & Horridge, 1965; Hyman, 1940). However, subsequent molecular phylogenetic analyses have removed the flatworms from this basal position and placed the entire flatworm phylum within the Lophotrochozoa, one of the two protostome superclades (Adoutte, Balavoine, Lartillot, & De Rosa, 1999; Adoutte et al., 2000). From this molecular phylogenetic viewpoint, there is no reason to assume that the flatworm central nervous system is any more basal than that of the other lophotrochozoan animals. Current molecular phylogenomic studies have now actually split the flatworms into two widely separated clades, the platyhelminth flatworms, which remain embedded among the lophotrochozoan phyla, and the acoelomorph flatworms, which are placed either at the base of the bilaterians or associated with the deuterostomes either as basal deuterostomes or as the sister group of hemichordates and echinoderms (Hejnol et al., 2009; Mwinyi et al., 2010; Philippe, Brinkmann, Martinez, Riutort, & Baguñá, 2007; Philippe et al., 2011). Thus, depending on their precise phylogenetic position, the acoel (but not the platyhelminth) flatworms and their supposedly “primitive” nervous systems are either basal to all bilaterians or basal only to the deuterostomes or highly derived and related to hemichordate nervous systems.

Figure 6.1 Summary Scheme of the Metazoan Phylogeny.
It is widely agreed that the cnidarians are the sister clade to the bilaterian animals. Note that the former flatworm group has been split into the “Acoela” and the “Platyhelmithes” (Philippe et al., 2007). Whereas the Platyhelminthes remained embedded within the Lophotrochozoans, the phylogenetic position of the Acoela is still a matter of debate. The newest studies either place the Xenoturbellida, the Nemertodermatida, and the Acoela at the base of the Bilateria as a sister group to all other bilaterian animals (Hejnol et al., 2009; Mallat, Craig, & Yoder, 2010), at the base of the Deuterostomes, or within the Deuterostomes (Philippe et al., 2011).
Third, it has been found to be very difficult to consider the nervous systems of extant animal groups as primitive, even when they are located on a very basal position within the tree of life. It is widely, but not universally, agreed that Porifera, Cnidaria, Ctenophora, and Placozoa are basal animal groups. Since sponges and placozoans have neither nervous systems nor neurons, they are of limited help in defining the first nervous system. Neurons and nervous systems are present in Cnidaria and Ctenophora, as well as in all other eumetazoan animals, and therefore it has been hypothesized that the first nervous system evolved after the evolutionary separation of the Porifera from the Radiata (reviewed in Lichtneckert & Reichert, 2007). Since this implies that the Cnidaria might be the most basally branching phylum of the Eumetazoa manifesting a nervous system, the nervous organization of these animals has been studied in some detail. These studies show that cnidarian nervous systems are remarkably diverse, ranging from diffuse nerve nets, in which there is little central integration and the sensory input and motor output are processed locally, to clearly centralized nervous systems with ganglion‐like nervous centers that are associated with sophisticated sensory organs such as lens eyes (Satterlie, 2011). It is largely arbitrary to consider any one of the diverse nervous system types to be basal and hence “primitive” in this phylum, since even the diffuse nerve‐net‐like nervous system type might represent the secondary loss of a previously present centralized nervous system.
In view of the problems in elucidating the origin of the nervous system based on classical comparative neuroanatomical analysis, a number of investigations in the last two decades have explored a novel approach to nervous system evolution that combines comparative studies with developmental and molecular genetic analysis. This new integrated approach has revealed considerable insight into the evolutionary origin of the brain and central nervous system of bilaterian animals. Moreover, it has provided new insight into the origin of centralized nervous systems that may also be relevant for understanding the origin of the first metazoan nervous system.
6.3 The First Bilaterian Nervous System
6.3.1 Diversity of Bilaterian Nervous Systems
Many different morphological types and shapes of nervous systems are found in extant bilaterians. All deuterostomes investigated have central nervous systems and peripheral nervous systems. The peripheral nervous systems can be highly variable in structure ranging from the nerve‐net type of organization seen in the vertebrate enteric nervous system to the ordered ganglionic organization exemplified by the vertebrate autonomic nervous system. The central nervous system of deuterostome chordates is, in general, less variable in structure. In the chordates, which include the vertebrates, the central nervous system comprises an anterior brain, subdivided into multiple compartment‐like substructures, that is associated with sensory organs and is connected to a dorsally located nerve cord which links the brain to the peripheral body parts. In the urochordate tunicates, this type of central nervous system organization is only present in the larva and is radically reduced after metamorphosis in the sedentary adult form. In cephalochordates, the subdivisions in the brain and nerve cord are cryptic but can be revealed with molecular markers (Nieuwenhuys, 2002).
The central nervous systems of the remaining deuterostome phyla are more diverse. Hemichordates, which have been thought to posses only a net‐like peripheral nervous system, are now known to have a fully formed central nervous system comprising dorsal as well as ventral nerve cords (Lowe et al., 2003; Nomaksteinsky et al., 2009; reviewed in Benito‐Gutierrez & Arendt, 2009; Holland et al., 2013). Echinoderms also possess central nervous systems, which are, however, clearly divergent from those of other deuterostomes due to the secondary acquisition of radial symmetry in these animals (Nieuwenhuys, 2002). Acoel flatworms (whether they are bona‐fide deuterostomes or not) and probably the flatworm‐like xenoturbellids have a central nervous system comprising an anterior ganglion and multiple nerve cords (Achatz & Martinez, 2012; Bullock & Horridge, 1965; Semmler, Chiodin, Bailly, Martinez, & Wanninger, 2010).
Most protostomes also have both central nervous systems and peripheral nervous systems. Prominent among the central nervous systems are the complex multiganglionic brains and nerve cords of most free‐living arthropods, annelids, and molluscs culminating in the remarkably complex brain of cephalopods. These complex nervous systems can be markedly reduced or absent in sedentary or parasitic forms within these and other protostome phyla. Central nervous systems with a somewhat more simple organization, consisting of an anterior ganglion and associated nerve cords, are seen in free‐living members of phyla as diverse as platyhelminth flatworms, ribbon worms, tardigrades, chaetognathes, sipunculids, rotifers, ectoprocts, and nematodes (Bullock & Horridge, 1965, Kotikova & Raiikova, 2008). As in deuterostomes, the peripheral nervous systems of protostomes can be highly variable in structure ranging from a diffuse nerve‐net type, seen in molluscs, to the ganglionic organization of some of the components of the arthropod peripheral nervous system.
Taken together, these data support the notion implied by current paleontological findings that centralized nervous systems were present in ancestral bilaterians before the protostome–deuterostome split. Moreover, based on comparative neuroanatomical data, these ancestral bilaterian central nervous systems likely consisted of an anterior brain‐like ganglion (“protobrain”) connected to descending nerve cord‐like structures (“protocords”) which may or may not have had ganglionic features (Ghysen, 2003). What is not at all obvious from these data is whether the diverse central nervous systems of extant bilaterians evolved separately or if they all had a common urbilaterian origin.
In its ontogeny, the bilaterian central nervous system is a complex three‐dimensional structure that develops from a two‐dimensional embryonic neuroepithelium. Since this neuroepithelium is located dorsally in most deuterostomes and ventrally in most protostomes, an independent evolutionary origin of the central nervous system in these two animal groups has been postulated (the gastroneuralia–notoneuralia concept; e.g., Brusca & Brusca, 1990). However, more recently (and supporting earlier ideas) the notion that the central nervous systems of protostomes and deuterostomes are homologous and derive from a common ancestral (urbilaterian) brain has been put forward (Arendt & Nübler Jung, 1994; Ghysen, 1992; Reichert & Simeone, 2001). This notion has received considerable support from the astounding conservation of developmental mechanisms that pattern the anteroposterior and dorsoventral axes of the central nervous system in several vertebrate and invertebrate model systems, as well as from the remarkable similarities in developmental origins of neuronal cell types and complex circuitry in bilaterian central nervous systems. Taken together, these recent comparative developmental genetic data indicate that similar mechanisms operate in many major stages of central nervous system formation in vertebrates and invertebrates, implying a monophyletic origin of the centralized bilaterian nervous system (Arendt et al., 2008; Hirth, 2010; Reichert, 2009).
6.3.2 Conserved Mechanisms for Anteroposterior Patterning of the Bilaterian Central Nervous System
During early development, the two‐dimensional neuroepithelium that gives rise to the neurons of the bilaterian central nervous system is subdivided into compartment‐like domains along both its axes by the regionalized expression of patterning genes. These genes and their respective patterns of expression are comparable in vertebrates and invertebrates. Patterning along the anteroposterior axis involves the cephalic gap genes, which are expressed in the anterior brain, the (homeotic) Hox genes, which are expressed in the posterior brain and nerve cord, and a set of other genes which delimit specific compartment interfaces in the central nervous system (see Figure 6.2).

Figure 6.2 Simplified Summary Scheme of the Anteroposterior Order of Conserved Gene Expression in Embryonic CNS Development of Bilaterians.
Dorsoventral patterning is not indicated. Schematic diagram shows the expression of the patterning genes optix/Six3, otd/Otx2, dFezf/Fezf, mirr/Irx, Pax 2/5/8, unpg/Gbx2 and Hox gene orthologues in the developing CNS of Drosophila and mouse. Expression domains are color‐coded. (left) Gene expression in Drosophila CNS of embryonic stage 14. Borders of the protocerebral, deutocerebral, tritocerebral, mandibular (s1), maxillary (s2), labial (s3), and ventral nerve cord (VNC) neuromeres are indicated by horizontal lines. (right) Gene expression in mouse CNS of embryonic day 9.5–12.5. Borders of the forebrain, midbrain and the hindbrain and its rhombomeres (r1‐r8) are indicated by horizontal lines. In both fly and mouse, an optix/Six3 expression domain patterns the most anterior CNS region and overlaps with the otd/Otx2 expression pattern (Steinmetz et al., 2010) which is anterior to the abutting unpg/Gbx2 expression (Bouillet, Chazaud, Oulad‐Abdelghani, Dollé, & Chambon, 1995; Urbach, 2007; Wassarman et al., 1997). In both animals, a Pax2/5/8‐ expression domain is positioned close to the interface between the anterior otd/Otx2 and the posteriorly abutting unpg/Gbx2 expression domains (Asano & Gruss, 1992; Hirth et al., 2003; Rowitch & McMahon, 1995). Hox genes orthologues expression follows posteriorly to the Pax2/5/8 expression domain in both animals (Davenne et al., 1999; Hirth et al., 1998; Lichtneckert & Reichert, 2007). Furthermore, the interface of the relative expression of dFezf/Fezf and mirr/Irx was reported to be conserved between fly and mouse (Irima et al., 2010; Oliver et al., 1995).
Adapted from Lichtneckert and Reichert, 2007.
Cephalic gap genes such as orthodenticle (otd)/Otx and empty spiracles (ems)/Emx encode transcription factors that were originally identified in the embryogenesis of the fruit fly, Drosophila, as key patterning elements for anterior cephalic domains (Cohen & Jurgens, 1990; Dalton, Chadwick, & McGinnis, 1989; Finkelstein & Perrimon, 1990). In addition to their role in head development, these genes are expressed in the anterior neuroectoderm of vertebrates and invertebrates and play key, evolutionarily conserved roles in central nervous system pattering (reviewed in Lichtneckert & Reichert, 2008). The most prominent of these is exemplified by otd/Otx which is expressed in the anterior brain or protobrain of bilaterians as diverse as planarians, nematodes, annelids, molluscs, arthropods, urochordates, cephalochordates, and vertebrates, including mammals (Acampora et al., 2001; Arendt, Technau, & Wittbrodt, 2001; Bruce & Shankland, 1998; Finkelstein, Smouse, Capaci, Spradling, & Perrimon, 1990; Hirth & Reichert, 1999; Lanjuin, VanHoven, Bargmann, Thompson, & Sengupta, 2003; Nederbragt, te Welscher, van der Driesche, van Loon, & Dictus, 2002; Schilling & Knight, 2001; Tomsa & Langeland, 1999; Umesono, Watanabe, & Agata, 1999; Wada, Saiga, Satoh, & Holland, 1998).
Functional studies carried out in fruit fly and mouse show that otd/Otx genes are required for formation and regionalization of the anterior neuroectoderm in both animals. Mutation of otd in Drosophila results in defective anterior neuroectoderm specification and failure in formation of stem‐cell like neuroblasts in this region (Hirth et al., 1995; Younossi‐Hartenstein et al., 1997). Mutation of Otx2, one of two otd homologs in mouse, results in lack of anterior brain structures due to an impairment in the specification of the anterior neuroectoderm (Acampora et al., 1995). The evolutionary conservation of expression and function of otd/Otx genes in anterior brain specification is underscored by cross‐phylum transgenic experiments in which the mammalian Otx genes were expressed in fly otd mutants and, inversely, in which Drosophila otd was expressed in mouse Otx mutants (Acampora et al., 1998; Acompora, Boyl et al., 2001; Acampora, Gulisano, Broccoli, & Simeone, 2001; Leuzinger et al., 1998). In both cases the transgene was able to effect a cross‐phylum rescue of brain development. Comparable cross‐phylum rescue experiments carried out for the ems/Emx genes, which are also regionally expressed in anterior brain regions of vertebrate and invertebrate bilaterians, showed that murine Emx1 can rescue brain defects in fly ems mutants (Hartmann, Hirth, Waldorf, & Reichert, 2000; Hirth et al., 1995). Interestingly, an Emx transgene from a non‐bilaterian cnidarian (Acropora) was not able to rescue the ems mutant brain defects of Drosophila, although it did rescue head patterning defects in the fly mutant (Hartmann et al., 2010).
Hox genes encode a set of evolutionarily conserved homeodomain transcription factors that are involved in the specification of regionalized identity during development (Carroll, 1995); their role in anteroposterior regionalization is thought to have evolved early in metazoan history (Finnerty, 2003). They are generally expressed along the developing anteroposterior body axis in the same order as their arrangement on chromosomes (“co‐linearity”). Hox gene expression is especially prominent in the developing central nervous system, which may be the ancestral site of Hox gene action in bilaterians (Hirth & Reichert, 2007). Hox genes are expressed in an ordered set of domains in the developing central nervous system of bilaterians as diverse as acoels, nematodes, annelids, molluscs, arthropods, urochordates, cephalochordates and vertebrates including zebra fish, chicken, mouse, and human (Carpenter, 2002; Hejnol & Martindale, 2009; Hirth & Reichert, 1999; Hughes & Kaufman, 2002; Hunt et al., 1991; Ikuta, Yoshida, Satoh, & Saiga, 2004; Irvine & Martindale, 2000; Kenyon et al., 1997; Kourakis et al., 1997; Lee, Callaerts, de Couet, & Martindale, 2003; Lumsden & Krumlauf, 1996; Moens & Prince, 2002; Steinmetz, Kostyuchenko, Fischer, & Arendt 2011; Vieille‐Grosjean, Hunt, Gulisano, Boncinelli, & Thorogood, 1997; Wada, Garcia‐Fernandez, & Holland, 1999; Wilkinson, Bhatt, Cook, Boncinelli, & Krumlauf, 1989).
Mutant analyses of Hox gene action in central nervous system development of fly and mouse reveal a comparable function in specification of regional identity. In Drosophila, Hox genes are required for the specification of regionalized neuronal identity in the posterior brain (Hirth, Hartmann, & Reichert, 1998). In mouse, Hox genes are involved in specifying the rhombomeres of the developing hindbrain (Gavalas et al., 1998; Studer, Lumsden, Ariza‐McNaughton, Bradley, & Krumlauf, 1996; Studer et al., 1998). This evolutionary conservation of Hox gene action in central nervous system development is emphasized by the fact that cis‐regulatory regions driving the specific spatiotemporal expression of Hox genes are interchangeable between insects and mammals (Malicki, Cianetti, Peschle, & McGinnis, 1992; Popperl et al., 1995). Together, these data imply that expression, function, and regulation of Hox gene action in central nervous system development are conserved features of this developmental control gene family.
While Hox genes are expressed in the posterior brain and nerve cord of bilaterians, they are excluded from the region of otd/Otx2 and ems/Emx gene expression in the anterior brain. In vertebrates, a marked boundary region in the developing brain called the midbrain–hindbrain boundary (MHB) is located anterior to the expression domain of the Hox genes, and this region has an essential organizer function in patterning the midbrain and anterior hindbrain (Liu & Joyner, 2001; Rhinn & Brand, 2001; Wurst & Bally‐Cuif, 2001). In vertebrates too, the developing MHB is delimited by the interface of the posterior Otx2 expression domain and an abutting Gbx2 expression domain, and it is also characterized by the expression of Pax2/5/8 encoding genes. In Drosophila, a comparable boundary region is found in the developing brain anterior to the Hox expression domain; this region is also delimited by the interface of the posterior otd/Otx2 domain and the abutting unplugged (unpg)/Gbx2 expression domain, and is similarly characterized by the expression of Pax2/5/8 (Hirth et al., 2003; Urbach, 2007). Comparable expression patterns of homologs of these genes are found anterior to the Hox expression domains in the developing brains of several other deuterostome and protostome taxa (Holland, 2009; Irimia et al., 2010; Steinmetz et al., 2011; Wada et al., 1998; Wada & Satoh, 2001). Hence, a defined boundary region between the anterior (otd/Otx2‐expressing) and the posterior (Hox‐expressing) parts of the brain, which together have been considered to be representative of a tripartite organization of the ancestral chordate brain, appears to be evolutionarily conserved in bilaterians.
In vertebrate brains, a second region with organizer function is found at the zona limitans intrathalamica (ZLI) which develops within the diencephalon at the boundary between the expression domains of the Fezf and the Irx genes (Irimia et al., 2010). Comparable patterns of abutting gene expression define a ZLI‐like boundary zone in the anterior brain of the basal chordate Amphioxus, implying that a ZLI‐like structure is a conserved feature of chordate brains. Remarkably, a boundary of expression of the homologous insect genes is found in the anterior brain of Drosophila, where expression of dFezf, restricted to the anterior part of the brain, and expression of mirr, the earliest expressed fly Irx gene, adjoin to form a gene expression boundary (Irimia et al., 2010). The conserved nature of this ZLI‐like interface of gene expression domains provides further support for the conserved nature of brain development in bilaterians. Additional support for this notion is provided by the conserved expression of optix/Six3 genes in a comparable domain at the most anterior tip of the central nervous system neuroectoderm in animals as diverse as vertebrates, insects, and annelids (Oliver et al., 1995; Steinmetz et al., 2010). Thus the Six3‐Otx2 brain patterning system, like the Fezf‐Irx and Otx2‐Gbx patterning systems, may also be universal to central nervous system development in bilaterians.
It is noteworthy that comparable anteroposterior patterns of expression in a set of homologous genes are found in the net‐like peripheral nervous system of the hemichordate Saccoglossus (Lowe et al., 2003). Whether this is also true for the developing central nervous system of this hemichordate is not currently known (Nomaksteinsky et al., 2009).
6.3.3 Conserved Mechanisms for Dorsoventral Pattering of the Bilaterian Central Nervous System
In addition to its anteroposterior axis, the neuroectoderm also has a second axis, which can be considered either mediolateral or dorsoventral, since the plate‐like neuroectoderm can extend in a dorsal direction or can give rise to a neural tube through invagination. A second set of patterning genes subdivides the neuroectoderm along this dorsoventral axis in a manner that is conserved in vertebrates and invertebrates. Key among these is a set of homeobox genes, referred to as the columnar genes, which control the formation of longitudinal domains in the neuroectoderm. Moreover, the induction of the neuroectoderm that gives rise to the central nervous system appears to rely on conserved dorsoventral patterning mechanisms that determine the dorsoventral body axis itself as well as the location of the neuroectoderm along that axis (see Figure 6.3).

Figure 6.3 Schematic Representation of Examples of Conserved Dorsoventral Genetic Expression Boundaries in a Segmental Part of the Neuroectoderm in Arthropods (Left), Vertebrates (Middle) and Annelids (Right).
The vertebrate neuroectoderm is shown before folding. Anteroposterior patterning is not indicated. The neurogenic region is patterned in a dorsoventral fashion by a set of conserved patterning genes in all three animals, here indicated by color code. Note that the neuroectoderm of each animal is subdivided in two parts at its midline by a black vertical line enabling to show normally overlapping gene expression domains more clearly. At the bottom of the bars the overlap is shown for better comprehension. Within this overlay conserved neuron cell types emerging from this particular region are indicated by different circles (Arendt et al., 2008; Denes et al., 2007; Mizutani & Bier, 2008). The homologous proteins Dpp/ BMP4/ Bmp2/4 (violet) form a dorsoventrally inverted gradient in vertebrates with respect to Drosophila melanogaster and Platynereis dumerilii. In Drosophila and vertebrates, another homologous protein pair, namely Sog/ Chordin (brown) forms an opposing gradient with respect to the Dpp/ BMP4 pattern, where it inhibits Dpp/ BMP4 and therefore enables induction of neurogenesis and with different gradients gives identity to different subdomains of the neuroectoderm (Lichtneckert & Reichert, 2005). The dorsoventral columnar patterning genes are highly conserved between the bilaterian animals (see comparable relative expression domains of vnd/ Nkx2.2/ nkx2.2 (yellow), ind/ Gsh/ gsh (orange), msh/ Msx1/msx (red), Nkx6.1+Nkx6.2/ nkx6 (light green) in Drosophila, mouse and Platynereis) (Lichtneckert & Reichert 2007; Seibert, Volland, & Urbach, 2009). In the annelid and the mouse neuroectoderm even more similarities compared to Drosophila are apparent, such as the additional Dbx1/2/ dbx and Dlx/ dlx expression domains, the columnar medial Pax6 expression (red dots) domain (Mizutani & Bier, 2008), as well as the Pax3/7 expression which in Drosophila is expressed in a strictly segmented fashion (dark green) (Denes et al., 2007).
A set of conserved interacting signaling molecules play key roles in the establishment of dorsoventral polarity during embryogenesis. Central among these are morphogen‐like signaling molecules of the Transforming Growth Factor β (TGFβ) family, represented by BMP4 in vertebrates and its homolog Dpp in Drosophila (De Robertis, 2008; De Robertis & Sasai, 1996). This BMP signalling pathway appears to be conserved in dorsoventral polarity formation in bilaterian animals such as insects, spiders, vertebrates, amphioxus and annelids, with the exceptions of nematodes and tunicates, which both have a modified type of development (Akiyama‐Oda & Oda, 2006; Denes et al., 2007; Levine & Brivanlou, 2007; Little & Mullins, 2006; Lowe et al., 2006; Miya, Morita, Ueno, & Satoh, 1996; Mizutani et al., 2005, Mizutani, Meyer, Roelink, & Bier 2006; Sasai, Lu, Steinbeisser, & De Robertis, 1995; Suzuki et al., 1999; Yu et al., 2007). The polarizing action of BMP4/Dpp is antagonized in a spatially restricted manner by a second group of conserved extracellular signaling molecules which include Chordin in vertebrates and its homolog Sog in Drosophila (Holley et al., 1995). The interacting Chordin/Sog and BMP4/Dpp signaling molecules act from opposing dorsoventral poles, and these poles are inverted in vertebrates versus invertebrates such as arthropods and annelids. (This provides strong support for the “dorsoventral inversion” hypothesis brought forward by Geoffroy Saint‐Hilaire (1822) which states that the dorsoventral axis of vertebrates and invertebrates are equivalent but inverted; see Arendt & Nübler‐Jung, 1994; De Robertis & Sasai, 1996). In addition to its polarizing function, the BMP4/Dpp morphogen suppresses development of the neuroectoderm and this suppressive function is inhibited by Chordin/Sog acting along the induced dorsoventral axis. Hence, in both vertebrate and invertebrate bilaterians, the region of the embryo that forms the neuroectoderm (dorsal in vertebrates, ventral in invertebrates) is the one in which Chordin/Sog is expressed and inhibits invading BMP4/Dpp. Indeed, whenever a central nervous system develops in vertebrates, insects, annelids and cephalochordates it derives from a neuroectoderm on the non‐BMP body side (Denes et al., 2007; Levine & Brivanlou, 2007; Mizutani et al., 2005, 2006; Sasai et al., 1995; Yu et al., 2007). This suggests that the functional conservation of the Chordin/Sog and the BMP4/Dpp morphogens in CNS neuroectoderm induction represents a conserved dorsoventral patterning mechanism that was already present in the urbilaterian ancestor of vertebrates and invertebrates.
Following early neuroectoderm induction, a conserved set of homeodomain proteins, encoded by the vnd/Nkx2.2, ind/Gsh and msh/Msx1 genes, act in further dorsoventral regionalization of the developing CNS (Chan & Jan, 1999; Cornell & Von Ohlen, 2000). All three genes are expressed in specific, nonoverlapping longitudinal columnar domains along the dorsoventral (or mediolateral) axis of the central nervous system. In Drosophila, vnd is expressed in a ventral column, ind in an intermediate column, and msh in a dorsal column of the ventral neuroectoderm; in the mouse, Nkx2.2 is expressed in a ventral, Gsh in an intermediate, and Msx1 in a dorsal column of the neural tube (Briscoe et al., 1999; Chu, Parras, White, & Jiménez 1998; Hsieh‐Li et al., 1995; Isshiki, Takeichi, & Nose, 1997; McDonald, Holbrook, Isshiki, Weiss, Doe, Mellerick, 1998; Pabst, Herbrand, & Arnold, 1998; Pera & Kessel, 1998; Qui, Shimamura, Sussel, Chen, & Rubenstein 1998; Shimamura, Hartigan, Martinez, Puelles, & Rubenstein, 1995; Sussel, Marin, Kimura, & Rubenstein, 1999; Valerius et al., 1995; Wang, Chen, Xu, & Lufkin, 1996; Weiss et al., 1998). In both animals these so‐called columnar genes control the formation of corresponding columnar dorsoventral identity domains, and act in neurogenesis at their site of action. These findings suggest that the role of the columnar genes in dorsoventral patterning of the central nervous system might be conserved throughout bilaterians (reviewed in Arendt & Nübler‐Jung, 1999; Lichtneckert & Reichert, 2007; Reichert & Simeone, 2001; Urbach & Technau, 2008). In support of this idea, comparable longitudinal domains of expression of homologous columnar genes are observed in the neuroectoderm of the lophotrochozoan annelid Platynereis. Even more extensive similarities in putative dorsoventral patterning genes are seen in the annelid versus vertebrate neuroectoderm, in that a columnar Pax6 expression domain as well as a columnar lateral Pax3/7 expression domain is apparent in both animals (Briscoe, Pierani, Jessell, & Ericson, 2000; Denes et al., 2007; Ericson et al., 1997; Kriks, Lanuza, Mizuquchi, Nakafuka, & Goulding, 2005). (Pax3/7 is also expressed in the developing central nervous system of Drosophila
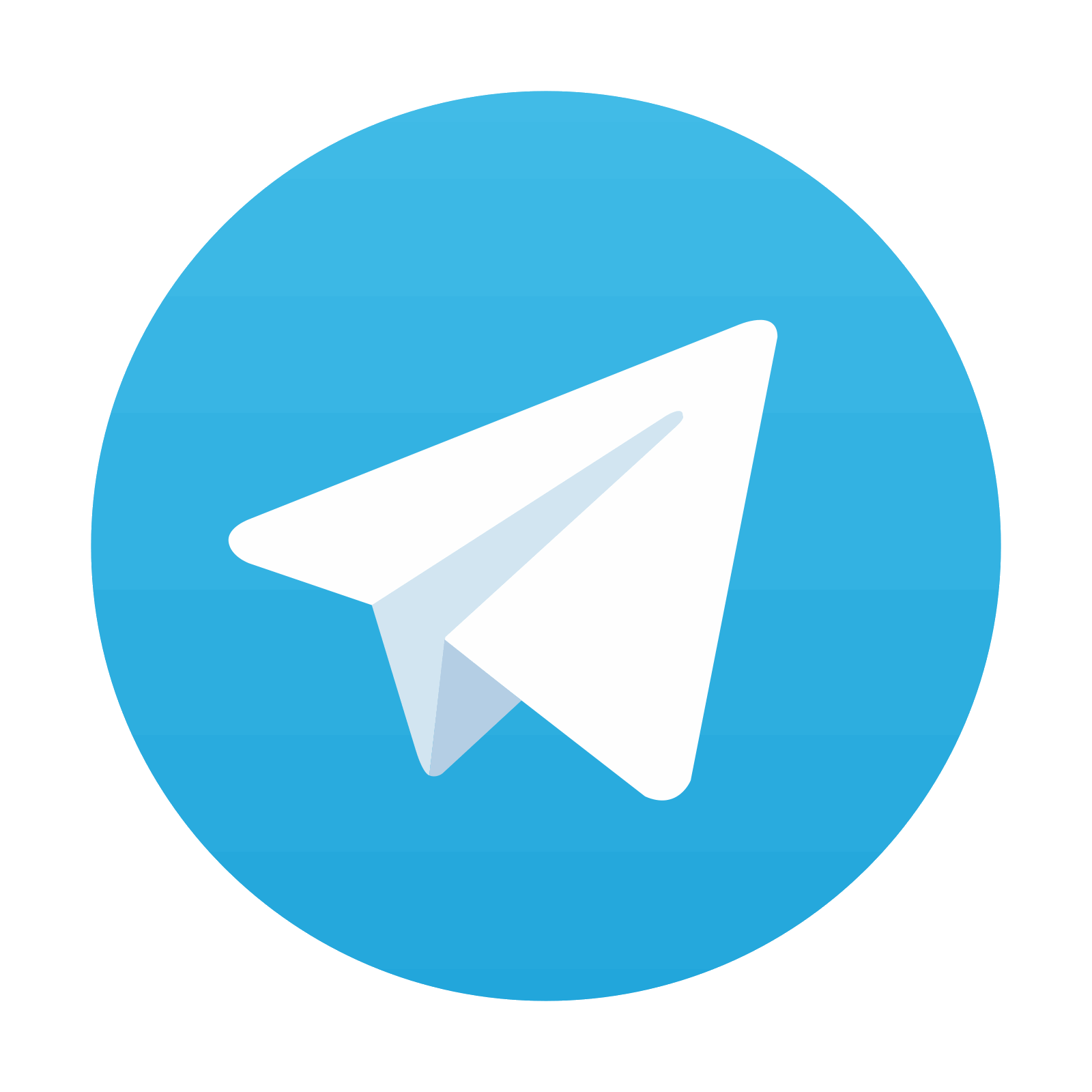
Stay updated, free articles. Join our Telegram channel
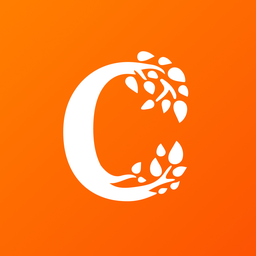
Full access? Get Clinical Tree
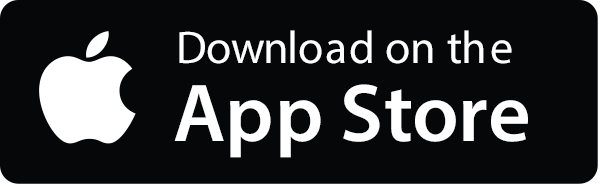
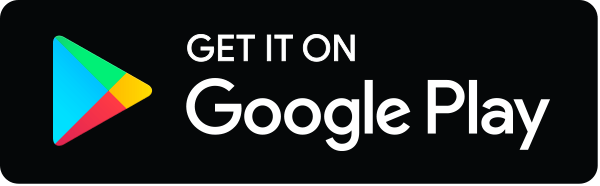