The New Neuroembryology
Harvey B. Sarnat
Preformation is represented by DNA, not by … a tiny adult in every sperm.
—Antonio García-Bellido, 1998 (1)
Traditional embryology is descriptive morphogenesis: detailed observations of gross and microscopic changes in organs and tissues in the developing embryo and fetus. The new neuroembryology is an integration of classic embryology with the insight into molecular genetic programs that direct cellular and regional differentiation to provide an explanation for the precise spatial and temporal sequences of these anatomic changes. Traditional embryology recognizes a series of developmental processes that are not entirely sequential because of a great deal of temporal overlap as the various processes proceed simultaneously. Several excellent recent textbooks effectively integrate descriptive morphogenesis and genetic programming (2,3,4). In human neuropathology, the gross and microscopic changes seen postmortem are temporally sequenced so precisely that they allow a precise correlation of brain maturation with gestational age and provide evidence of developmental delay in some cases (5).
By means of molecular genetics, the focus on the concepts of normal and abnormal development of the nervous system has shifted from traditional categories of structural change, such as neuronogenesis, cell migration, axonal projection, and synaptogenesis, to an understanding of preprogrammed mechanisms that allow these overlapping processes to proceed. Interactions of genes or their transcription products specify differentiation; developmental genes may be expressed only transiently in the embryo or continuously throughout life. After development appears complete, they conserve the identity of individual cellular types even in the adult and perhaps provide clues to degenerative processes that begin after the nervous system is mature.
The relevance of the new neuroembryology to clinical pediatric neurology, apart from new insights, is in its promise of new approaches for the prevention, and perhaps even potential treatment, of malformations of the nervous system. As a result of these new concepts, one must consider an entirely new and still largely unfamiliar classification of cerebral malformations. Such a classification is to be based on the defective expression or coexpression of transcription products of various developmental genes that determine and coordinate every aspect of neuroembryonic development, from gastrulation and the creation of a neuroepithelium to the last detail of postnatal synaptogenesis and myelination. Of additional importance is the understanding that the development of the nervous system no longer can be isolated from the rest of the embryonic and fetal body, because it is influenced by surrounding structures and also influences them: Segmentation of spinal nerve roots by mesodermal somites is an example of the former, and craniofacial development induced by neural crest migrating from the neural tube exemplifies the latter.
GASTRULATION
It is not birth, marriage, or death, but gastrulation which is truly the most important time in your life.
—Lewis Wolpert, 1978 (2)
Gastrulation is the birth of the nervous system. It is the time when a neuroepithelium may first be recognized as distinctive from other cells or tissues. In simple chordates, such as amphioxus and amphibians, gastrulation is the invagination of a spherical blastula. In birds and mammals, the blastula is collapsed as a flattened, bilayered disc, and gastrulation appears not as an invagination, but as a groove between two ridges on one surface of this disc, the primitive streak on the epiblast. The primitive streak establishes, in each embryo, the basic body plan of all vertebrates: a midline axis, bilateral symmetry, rostral and caudal ends, and dorsal and ventral surfaces.
As the primitive streak extends forward, an aggregate of cells at one end is designated the primitive node or Hensen’s node. Hensen’s node defines the rostral direction. Cells of the epiblast on either side move toward the primitive streak, stream through it, and emerge beneath it to pass into the narrow cavity between the two sheets of cells, the epiblast above and the hypoblast below. These migratory cells give rise to the mesoderm and endoderm internally, and some then replace the hypoblast (2).
After extending approximately halfway across the blastoderm (epiblast), the primitive streak with Hensen’s node reverses the direction of its growth to retreat, moving posteriorly as the head fold and neural plate form anterior to Hensen’s node. As the node regresses, a notochordal process develops in the area rostral to it and somites begin to form on either side of the notochord, the more caudal somites differentiating first and successive ones anterior to somites already formed. The notochord induces epiblast cells to form neuroectoderm (see the following Induction section).
INDUCTION
Experimental studies of the formation of the neural plate have yielded extraordinarily interesting information as the way one part of a developing embryo may influence the differentiation of other parts.
—B. M. Patten, 1951 (6)
Induction is a term denoting the influence of one embryonic tissue on another so that both the inducer and the induced differentiate as different mature tissues. In the case of the nervous system, the creation and subsequent development of the neural tube may be defined in terms of gradients of inductive influences.
Induction usually occurs between germ layers, as with the notochord (mesoderm) inducing the floor plate of the neural tube (ectoderm). Induction also may occur within a single germ layer, however. An example is the optic cup (neuroectoderm) inducing the formation of a lens and cornea from the overlying epithelium (surface ectoderm) that otherwise would have simply differentiated as more epidermis. Neural induction is the differentiation or maturation of structures of the nervous system from undifferentiated ectodermal cells because of the influence of surrounding embryonic tissues not of ectodermal origin. Neural induction is a term also used to describe the opposite: the effects of primitive neural tissues on the development of non-neural structures. An example is craniofacial development, in which neural crest cells migrating from the prosencephalon and mesencephalon of the neural tube induce the formation of membranous cranial and facial bone, cartilage, connective tissue, blood vessels, nerve sheaths, ocular globes, and many other tissues of the face and head (see the Neural Crest section later in this chapter).
Induction was discovered in 1924 by Hans Spemann and Hilde Mangold, who demonstrated that the dorsal lip of the newt gastrula was capable of inducing the formation of an ectopic second nervous system when transplanted to another site in a host embryo, in another individual of the same species, or in a ventral site of the same embryo (7). This dorsal lip of the amphibian gastrula (also known as the Spemann organizer) is homologous with Hensen’s node of embryonic birds and mammals. The transplantation of the primitive node in a similar manner as in the Spemann and Mangold experiments in amphibians yields similar results.
The first gene isolated from the Spemann organizer was Goosecoid (Gsc), a homeodomain protein (for definition, see Patterning of the Neural Tube: Axes and Gradients of Growth and Differentiation section) able to recapitulate the transplantation of the dorsal lip tissue. When injected into an ectopic site, Gsc also normally induces the prechordal mesoderm and contributes to prosencephalic differentiation (8,9,10). Another gene also expressed in Hensen’s node and even before the primitive streak is fully formed, Wnt-8C, also is essential for the regulation of axis formation and later for hindbrain patterning in the region of the future rhombomere 4 (see Segmentation section) (11). The regulatory gene Cnot, with major domains in the primitive node, notochord, and prenodal and postnodal neural plate, is another important molecular genetic factor responsible for the induction of prechordal mesoderm and for the formation of the notochord in particular (12). The gene (transcription factor) Gooseberry (Gox) is unique in being the only identified gene to be expressed in the primitive streak that loses expression at the onset of notochord formation. The Gox gene probably regulates the primitive streak either positively, by promoting its elongation, or negatively, by suppressing formation of the notochordal process. Another possible function is to promote a mesodermal
lineage of epiblast cells as they ingress through the primitive streak. Several other genes essential in creating the fundamental architecture of the embryo and its nervous system are already expressed in the primitive node (10), and many reappear later to influence more advanced stages of ontogenesis.
lineage of epiblast cells as they ingress through the primitive streak. Several other genes essential in creating the fundamental architecture of the embryo and its nervous system are already expressed in the primitive node (10), and many reappear later to influence more advanced stages of ontogenesis.
The specificity of induction is not the inductive molecule, but rather the receptor in the induced cell. This distinction is important because foreign molecules similar in structure to the natural inductor molecule may at times be recognized erroneously by the receptor as identical; such foreign molecules thus can behave as teratogens if the embryo is exposed to such a toxin. Induction occurs during a precise temporal window; the time of responsiveness of the induced cell is designated its competence, and it is incapable of responding either before that precise time or thereafter (13).
Induction receptors are not necessarily in or on the plasma membrane of the cell, but also can be in the cytoplasm or in the nucleoplasm. Retinoic acid (see Retinoic Acid section) is an example of a nuclear inducer. In some cases, the stimulus acts exclusively at the plasma membrane of target cells and does not require actual penetration of the cell (13,14). The receptors that represent the specificity of induction also are genetically programmed: A gene known as Notch is particularly important in regulating the competence of a cell to respond to inductive cues from within the neural tube and in surrounding embryonic tissues (15). Some mesodermal tissues, such as smooth muscle of the fetal gut, can act as mitogens on the neuroepithelium by increasing the rate of cellular proliferation (16,17), but this phenomenon is not true neural induction because the proliferating cells do not differentiate or mature. Some organizer and regulatory genes of the nervous system, such as Wnt-1, also exhibit mitogenic effects (18), and both insulin-like and basic fibroblast growth factors (bFGF) act as mitogens as well (19,20,21).
The early formation of the neural plate is not accomplished exclusively by mitotic proliferation of neuroepithelial cells, but also by a conversion of surrounding cells to a neural fate. In amphibians, a gene known as Achaete-scute (Xash-3) is expressed early in the dorsal part of the embryo from the time of gastrulation and acts as a molecular switch to change the fate of undifferentiated cells to become neuroepithelium rather than surface ectodermal or mesodermal tissues (22). Some cells differentiate as specific types because they are actively inhibited from differentiating as other cell types. All ectodermal cells are preprogrammed to form neuroepithelium, and neuroepithelial cells are preprogrammed to become neurons if not inhibited by genes that direct their differentiation along a different lineage, such as epidermal, glial, or ependymal (23,24,25).
SEGMENTATION
The primitive segmentation of the vertebrate brain is a problem which has probably attracted as much of the attention of morphologists as any one of the great, unsettled questions of the day, and many views have been advanced.
—C. F. W. McClure, 1890 (26)
Over a century ago, the concept of segmentation was already an issue actively debated by biologists who were intrigued by the repeating units along the rostrocaudal axis of many worms and insects as well as by the somites of embryonic vertebrates. Segmentation of the neural tube creates intrinsic compartments that restrict the movement of cells by physical and chemical boundaries between adjacent compartments. These embryonic compartments are known as neuromeres.
The spinal cord has the appearance of a highly segmented structure but is not intrinsically segmented in the embryo, fetus, or adult. Instead it corresponds in its entirety to the caudalmost of the eight neuromeres that create the hindbrain. The apparent segmentation of the spinal cord is caused by clustering of nerve roots imposed by true segmentation of surrounding tissues derived from mesoderm, tissues that form the neural arches of the vertebrae, the somites, and associated structures. Neuromeres of the hindbrain are designated rhombomeres (27,28,29,30). The entire cerebellar cortex, vermis, flocculonodular lobe, and lateral hemispheres, develops from rhombomere 1 with a small contribution from the mesencephalic neuromere, but the dentate and other deep cerebellar nuclei are formed in rhombomere 2 (31,32). The rostral end of the neural tube forms a mesencephalic neuromere and probably six forebrain neuromeres, as three diencephalic and three telencephalic prosomeres (33,34,35,36). The segmentation of the human embryonic brain into neuromeres is summarized in Table 5.1.
The segments of the embryonic neural tube are distinguished by physical barriers formed by processes of early-specializing cells that resemble the radial glial cells that appear later in development (37,38) and also by chemical barriers from secreted molecules that repel migratory cells. Cell adhesion is increased in the boundary zones between rhombomeres, which also contributes to the creation of barriers against cellular migration in the longitudinal axis (38). Limited mitotic proliferation of the neuroepithelium occurs in the boundary zones between rhombomeres. Although cells still divide in this zone, their nuclei remain near the ventricle during the mitotic cycle and do not move as far centrifugally within the elongated cell cytoplasm during the interkinetic gap phases as they do in general (38). The rhombomeres of the brainstem
also may be visualized as a series of transverse ridges and grooves on the dorsal surface, the future floor of the fourth ventricle; these ridges are gross morphologic markers of the hindbrain compartments (26,30).
also may be visualized as a series of transverse ridges and grooves on the dorsal surface, the future floor of the fourth ventricle; these ridges are gross morphologic markers of the hindbrain compartments (26,30).
TABLE 5.1 Segmentation of the Neural Tube | ||||||||||||||||||||||||||||||
---|---|---|---|---|---|---|---|---|---|---|---|---|---|---|---|---|---|---|---|---|---|---|---|---|---|---|---|---|---|---|
|
The first evidence of segmentation is a boundary that separates the future mesencephalic neuromere from rhombomere 1 of the hindbrain. More genes play a role in this initial segmentation of the neural tube than in the subsequent formation of other boundaries that develop to separate other neuromeres. Furthermore, the mesencephalic–metencephalic region appears to develop early as a single independent unit or organizer for other neuromeres rostral and caudal to that zone (39,40). The organizer genes recognized at the mesencephalic–metencephalic boundary for this earliest segmentation of the neural tube include Pax-2, Wnt-1, En-1, En-2, Pax-5, Pax-8, Otx-1, Otx-2, Gbx-2, Nkx-2.2, and FGF-8.
The earliest gene known with regional expression in the mouse is Pax-2, which is expressed even before the neural plate forms. It is the earliest gene recognized in the presumptive region of the midbrain–hindbrain boundary (41,42). In invertebrates, Pax-2 is important for the activation of Wingless (Wg) genes; this relationship is relevant because the first gene definitely associated with an identified midbrain–hindbrain boundary in vertebrates is Wnt-1, a homologue of Wg. The regulation of Wnt-1 may be divided into two phases: In the early phase (1 to 2 somites), the mesencephalon broadly expresses the gene throughout. In the later phase (15 to 20 somites), expression is restricted to dorsal regions, the roof plate of the caudal diencephalon, mesencephalon and myelencephalon, and spinal cord. It also is expressed in a ring that extends ventrally just rostral to the midbrain–hindbrain boundary and in the ventral midline of the caudal diencephalon and mesencephalon (43,44,45). Wnt-1 is essential in activating and preserving the function of the Engrailed genes En-1 and En-2. En-1 is coexpressed with Wnt-1 at the one-somite stage, in a domain only slightly caudal to Wnt-1, which includes the midbrain and rhombomere 1, the rostral half of the pons, and the cerebellar cortex but excludes the diencephalon (46). The activation of En-2 begins at the four-somite stage, and its function in mesencephalic and rhombomere 1 development is similar, with differences in some details, particularly their roles in cerebellar development (47,48). Finally, the homeobox gene Otx-2 appears early in the initial boundary zone of the midbrain–hindbrain, and, as with Wnt-1, it appears to be essential for the later expression of En-1 and En-2 and also of Wnt-1 (49,50).
The creation of neuromeres allows the development of structures within regions of the brain without the neuroblasts that form these nuclei wandering to other parts of the neuraxis where they would not be able to later establish their required synaptic relations. The interaction of genes with one another is a complexity that makes analysis of single-gene expression more difficult in interpreting programmed malformations of the brain.
PATTERNING OF THE NEURAL TUBE: AXES AND GRADIENTS OF GROWTH AND DIFFERENTIATION
Basic characteristics of the body plan are called patterning (27). They are the anatomic expression of the genetic code within the nuclear DNA of every cell, but they also may result from signals from neighboring cells, carried by molecules that are secretory translation products of various families of organizer genes, each in a highly precise and predictable temporal and spatial distribution.
The early development of the central nervous system (CNS) of all vertebrates, even before the closure of the
neural placode or plate to form the neural tube, requires the establishment of a fundamental body plan of bilateral symmetry, cephalization, or the identity of head and tail ends, and dorsal and ventral surfaces. This fundamental architecture of the body and of the neural tube requires the establishment of definite axes of growth and gradients of genetic expression and differentiation: (a) a longitudinal axis with rostrocaudal and caudorostral gradients, (b) a vertical axis with dorsoventral and ventrodorsal gradients, and (c) a horizontal axis with mediolateral and lateromedial gradients. Both normal ontogenesis and abnormal development of the embryonic nervous system involve these axes and gradients and should be put into this perspective (51).
neural placode or plate to form the neural tube, requires the establishment of a fundamental body plan of bilateral symmetry, cephalization, or the identity of head and tail ends, and dorsal and ventral surfaces. This fundamental architecture of the body and of the neural tube requires the establishment of definite axes of growth and gradients of genetic expression and differentiation: (a) a longitudinal axis with rostrocaudal and caudorostral gradients, (b) a vertical axis with dorsoventral and ventrodorsal gradients, and (c) a horizontal axis with mediolateral and lateromedial gradients. Both normal ontogenesis and abnormal development of the embryonic nervous system involve these axes and gradients and should be put into this perspective (51).
These axes of the body itself, and of the CNS, require the expression of genes that impose the gradients of differentiation and growth. The genes that determine the polarity and gradients of the anatomic axes are called organizer genes. Many express themselves not only in the CNS but in other organs and tissues as well (2,45). The bilateral symmetry of many organs and programmed asymmetries, probably including such neural structures as the different targets of the left and right vagal nerves and left–right asymmetries in the cerebral cortex, is determined in large part by Pitx-2, a gene expressed as early as in the primitive node (52).
The RNA that represents a transcript of the DNA translates a peptide, a glycoprotein, or some other molecule that acts to induce or at times to serve as a growth factor. Some genes also function to stimulate or inhibit the expression of others, or an antagonism or equilibrium can exist between certain families of genes, exemplified by those that exert a dorsoventral gradient and those that cause a ventrodorsal gradient.
The difference between an organizer gene and a regulator gene really lies in its function, and often the same gene subserves both roles at different stages of development. The definitions and programs of these two groups are summarized in Tables 5.2 and 5.3.
Evolutionary Conservation of Genes
The sequences of nucleotides of DNA are so primordial to animal life that identical or nearly identical base pairs exist not only in all vertebrates, but in all invertebrates as well, from the simplest worms to the most complex primates (53). The evolutionary biologists of the nineteenth century, such as Charles Darwin and Thomas Huxley, were constantly searching for “the missing links” between mammals and other vertebrates, between humans and other primates, and, most of all, between invertebrates and vertebrates because comparative anatomy did not provide a sufficiently satisfactory answer. They never found the missing link because the technology of their day could not elucidate this fundamental and profound question. The missing link sought by the nineteenth-century evolutionary biologists lies in the identical sequences of nucleic acid residues that form the same organizer genes in all animals, from the simplest worms to humans.
TABLE 5.2 Programs of Developmental Genes | |
---|---|
|
Despite evolutionary conservation of genes, there may be some variability among species in the compartmental site within the embryonic neural tube of structures programmed by these genes. For example, the abducens motor nucleus forms in rhombomere 6 in elasmobranchs (sharks) but in rhombomere 5 in mammals; the trigeminal/facial boundary shifts from rhombomere 4 in lampreys to rhombomere 3 in birds and mammals.
Transcription Factors and Homeoboxes
The genes that program the development of the nervous system are specific series of DNA base pairs linked to small proteins called transcription factors. These transcription factors are essential for the functional expression of these genes. A frequent transcription factor is the basic helix-loop-helix structure. It is so fundamental to the evolution of life that it appears for the first time in certain bacteria, even before a cell nucleus evolved to concentrate the DNA (53).
The zinc finger is another DNA-binding, gene-specific transcription factor. It consists of 28 amino acid repeats with pairs of cysteine and histidine residues, each sequence folded around a zinc ion (54). Krox-20 (this gene name is applied to the mouse; in the human it is redesignated EGR2) is a zinc finger gene expressed in alternating rhombomeres, especially rhombomere 3 and rhombomere 5; neural crest tissue does not migrate from those two rhombomeres (see Neural Crest section). Krox-20 also
serves an additional function in the peripheral nervous system, where it regulates myelination by Schwann cells (55). Finally, Krox-20 regulates the expression of some other genes, most notably those of the Hox family (56,57,58,59,50). Examples of other zinc fingers include Zic, TFIIIA, cSnR (snail related), and PLZF (human promyelocytic leukemia zinc finger) (61).
serves an additional function in the peripheral nervous system, where it regulates myelination by Schwann cells (55). Finally, Krox-20 regulates the expression of some other genes, most notably those of the Hox family (56,57,58,59,50). Examples of other zinc fingers include Zic, TFIIIA, cSnR (snail related), and PLZF (human promyelocytic leukemia zinc finger) (61).
TABLE 5.3 Organizer and Regulator Genes of the Embryonic and Fetal Nervous System | ||||||||||||||||||||||||||||||||||||||||||||||||||||||||||||||||||||||||||||||||||||||||||||||||||||||||||||||||||||||||||||||||||||||||||||||||||||||||||||||||||||||||||||||||||||||||||||||||||||||||||||||||||||||||||||||||||||||||||||||||||||||||||||||||||||||||||||
---|---|---|---|---|---|---|---|---|---|---|---|---|---|---|---|---|---|---|---|---|---|---|---|---|---|---|---|---|---|---|---|---|---|---|---|---|---|---|---|---|---|---|---|---|---|---|---|---|---|---|---|---|---|---|---|---|---|---|---|---|---|---|---|---|---|---|---|---|---|---|---|---|---|---|---|---|---|---|---|---|---|---|---|---|---|---|---|---|---|---|---|---|---|---|---|---|---|---|---|---|---|---|---|---|---|---|---|---|---|---|---|---|---|---|---|---|---|---|---|---|---|---|---|---|---|---|---|---|---|---|---|---|---|---|---|---|---|---|---|---|---|---|---|---|---|---|---|---|---|---|---|---|---|---|---|---|---|---|---|---|---|---|---|---|---|---|---|---|---|---|---|---|---|---|---|---|---|---|---|---|---|---|---|---|---|---|---|---|---|---|---|---|---|---|---|---|---|---|---|---|---|---|---|---|---|---|---|---|---|---|---|---|---|---|---|---|---|---|---|---|---|---|---|---|---|---|---|---|---|---|---|---|---|---|---|---|---|---|---|---|---|---|---|---|---|---|---|---|---|---|---|---|---|---|---|---|---|---|---|---|---|---|---|---|---|---|---|---|
|
Complexes of retinoic acid with its intranuclear receptor form still other transcription factors important in nervous system development, both normal and abnormal (see Retinoic Acid section). A unique transcription factor with coactivation domain requirements, Pit, programs anterior pituitary differentiation (62,63).
Growth factors, which also are molecules created by DNA sequences, are other influences on the establishment of the plan of the neural tube. Biologically, they act as transcription factors: bFGF behaves as an auxiliary inductor of the longitudinal axis with a rostrocaudal gradient during the formation of the neural tube (64).
Some transcription factors include homeoboxes. These are restricted DNA sequences of 183 base pairs of nucleotides that encode a class of proteins sharing a common or similar 60-amino acid motif termed the homeodomain (27). Homeodomains contain sequence-specific DNA-binding activities and are integral parts of the larger regulatory proteins, the transcription factors. Homeoboxes or homeotic genes are classified into various families with common molecular structure and similar general expression in ontogenesis. Homeoboxes are associated especially with genes that program segmentation and rostrocaudal gradients of the neural tube. Some of the important families of homeobox genes in the development of the
vertebrate nervous system are Gsc, Hox, En, Wnt, Shh, Nkx, LIM, and Otx.
vertebrate nervous system are Gsc, Hox, En, Wnt, Shh, Nkx, LIM, and Otx.
Families of Developmental Genes of the Central Nervous System
The genes that program the axes and gradients of the neural tube may be classified as families by their similar nucleic acid sequences and also by their similar general functions, although important differences occur within a family in the site or neuromere where each gene is expressed and in the anatomic structures they form. A dorsalizing gene not only has a dorsal territory of expression, but also causes the ventral parts of the neural tube to differentiate as dorsal structures if influences from ventralizing genes do not antagonize them with sufficient strength, and vice versa. A good example is the development of the somite. The sclerotome, which forms cartilage and bone of the vertebral body, normally is situated ventral to the myotome, which forms muscle cells, and the dermatome. Ectopic cells of the floor plate or of the notochord implanted next to the somite of the chick embryo cause a ventralization of the somite, so that excess cartilage and bone are formed and a deficiency of muscle and dermis occurs (65,66). The floor plate or notochord, in this instance, is the ventralizing inductor of the mesodermal somite, and it is now known that the genetic factor responsible is the transcription product of the gene Sonic hedgehog (Shh), which also serves as a strong ventralizing gradient force in the neural tube (25,67,68,69,70). If a section of notochord is implanted ectopically dorsal or lateral to the neural tube, a second floor plate forms opposite the notochord and motor neurons differentiate on either side of it, despite the presence of a normal floor plate and motor neurons in the normal position (71,72). Shh, a strong gene of the ventrodorsal gradient that becomes expressed as early as in the primitive node, has induced the ventralization of a dorsal region of the neural tube or duplicates the neural tube. Such an influence in the human fetus, the so-called split notochord, could be an explanation of the rare cases of diplomyelia or diastematomyelia (73). Excessive Shh, particularly its N-terminus cleavage product, upregulates floor plate differentiation at the expense of motor neuron formation (68) and might even induce duplication of the neuraxis. Furthermore, Shh also exerts a strong influence on the differentiation of ventral and medial structures of the prosencephalon (74), and the defective expression resulting from a mutation of this gene is thought to be the molecular basis of the human malformation holoprosencephaly (75).
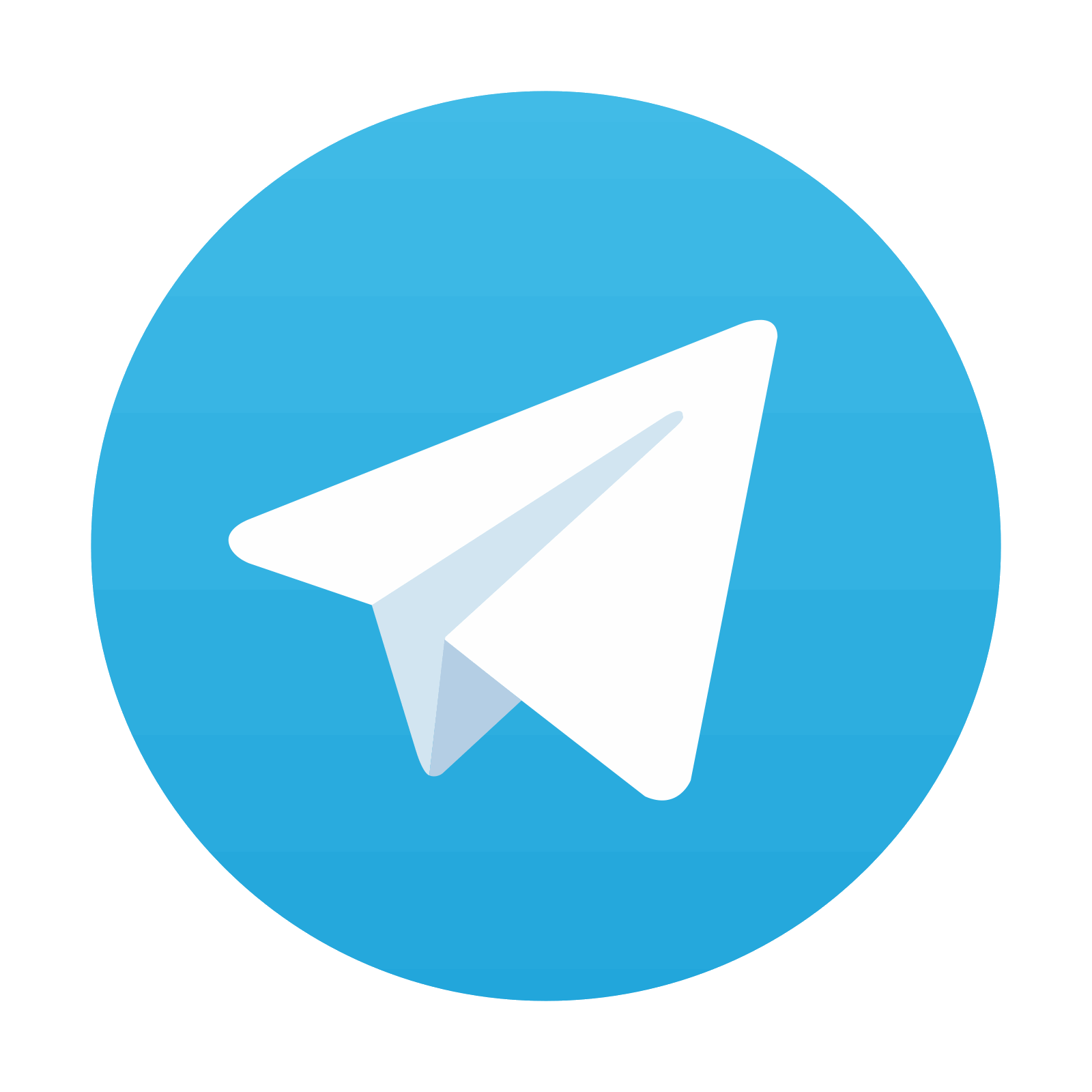
Stay updated, free articles. Join our Telegram channel
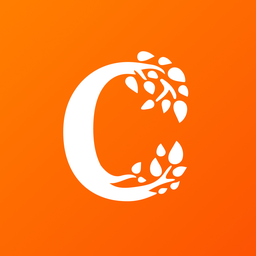
Full access? Get Clinical Tree
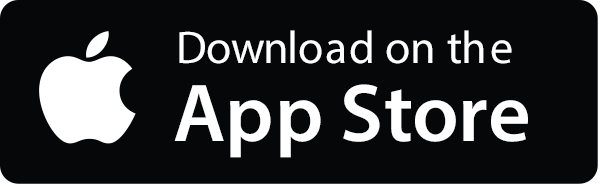
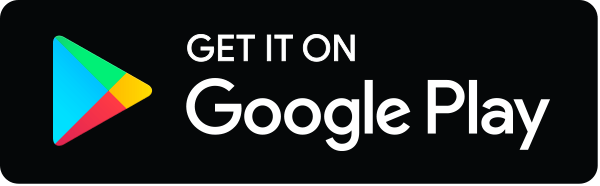