Abstract
Deep brain stimulation (DBS) of subcortical nodes improves the motor function in Parkinson disease, dystonia and tremor, and behavioral symptoms in obsessive-compulsive disorder. This resetting of dysfunctional brain networks by therapeutic DBS is mediated by white matter stimulation and modulation of network dynamics by adjusting local field potential. In parallel with investigations into novel indications, significant advancements in neurosurgical technique and stimulation titration have been reported. This chapter focuses on these advancements in techniques and technology, especially in the areas of neuroimaging and biomedical engineering intended to improve targeting and programming.
Keywords
Deep brain stimulation, Neuroimaging, Stimulation titration, Targeting, Techniques, Technological advances
Outline
Introduction 477
Precise Identification of Targets and Improved Stereotactic Targeting 478
Indirect Targeting 478
Direct Targeting and Its Implications 478
Substrates of DBS Efficacy and the Origin of Connectivity-Based Targeting 479
Stimulation Titration For Modulation of Dysfunctional Networks With DBS 480
Conclusions 482
References 482
Introduction
Deep brain stimulation (DBS) is an established treatment for movement disorders with distinct advantages over best medical therapy and lesioning procedures ( ). In Parkinson disease (PD), for example, in addition to improving motor symptoms, DBS improves the overall quality of life ( ) and is more cost effective than medical therapy alone in younger patients ( ). While the efficacy of lesioning (thalamotomy) may eventually fade, the benefits of DBS can be sustained for several years in refractory essential tremor (ET) ( ). Both subthalamic nucleus (STN) ( ) and globus pallidus interna (GPi) ( ) are equally efficacious, and the choice of one over the other may be tailored to patient-specific indications (e.g., GPi may be more suited for patients with PD with significant axial symptoms and dyskinesias). DBS also appears to be more efficacious than medical therapy in patients with PD with early motor complications ( ). Finally, the safety profile of DBS has been excellent with only 1%–2% the risk of serious complications after surgery ( ).
Despite this proven track record, only 10%–15% of eligible patients eventually undergo DBS; for example, with 10 million patients with ET ( ) and 1 million patients with PD ( ) living in the United States, only 5000 DBS procedures are performed annually ( ). Patient perceptions against invasive surgery and conservative approach of referring providers may be important drivers for this low utilization rate. It is also important to recognize that DBS has inherent limitations for the treatment of complex and progressive neurodegenerative disorders; despite an excellent motor efficacy, bilateral STN stimulation does not prevent falls and may be associated with adverse effects like dysarthria, paresthesias, and fatigue ( ). Loss of DBS efficacy in ET maybe a concern with one study reporting waning benefits in 73% of the patients after 18 months ( ). Although efficacy is satisfactory for primary generalized primary dystonia and DYT1 mutation, the results are less satisfactory in patients with secondary generalized dystonia ( ). Finally, DBS use for psychiatric applications has met with less-than-expected outcomes. Despite an established role of subgenual cingulate in the pathogenesis of major depressive disorder (MDD) ( ) and a promising pilot study, the pivotal multicenter trial was negative based on a predetermined improvement in primary outcome variable. Another large trial of DBS for depression was halted after a futility analysis ( ).
With increasing understanding of neural substrates of efficacious stimulation and the recognition of DBS-mediated modulation of brain networks, novel techniques and technologies are being used to address some of these challenges. We classify these advances in two major categories: those directed toward precise identification of stereotactic targets and those aimed at improving the stimulation paradigms. We also discuss a road map for the integration of these advances in future efforts to address the major challenges in this field.
Precise Identification of Targets and Improved Stereotactic Targeting
Indirect Targeting
The major interest in the past 10 years has been concentrated on improving the accuracy of targeting since it is crucial for achieving good clinical outcomes ( ). For better target identification, several human brain atlases, both printed and electronic, were introduced ( ). These atlases were based on anatomy of few postmortem brains and therefore the content was difficult to map on the patient brain. More recently, deformable computerized brain atlases have been developed ( ). This has improved registration, but there are some limitations in regions with high spatial complexity (e.g., the gyral anatomy of the cortex) ( ). Nonlinear registration methods are still under investigation and have yet to be used for clinical application. Overall, though, the accuracy of atlas-based stereotactic targeting is limited by the intrinsic variability in the location of deep targets that are not distinctly visible on structural imaging ( ). compared the atlas-based VIM target with the location of the dentorubrothalamic tract (as determined by deterministic tractography) and concluded that the “optimal” implantation coordinates were more lateral than the planned atlas-based coordinates. Similarly, correlated the lead location in STN and GPi relative to MCP with the motor outcome in 96 patients with PD. The MCP-based lead location did not correlate with postoperative Unified Parkinson’s Disease Rating Scale (UPDRS) score variation. The authors concluded that the MCP-based coordinate could not capture the variability in individual anatomy and connectivity. Because microelectrode recording and testing represent the gold standard for intraoperative target confirmation ( ), creating probabilistic maps with physiologic and functional information appears to be the most comprehensive approach for developing targeting atlases ( ). For improving the targeting accuracy, intraoperative guidance with two-dimensional radiographs is most commonly used ( ). Additional emerging methods include O-Arm (Medtronic, Inc.) ( ) and intraoperative computed tomography ( ).
Direct Targeting and Its Implications
Interestingly, there is significant variability in targeting methods even among experienced neurosurgeons ( ). This may, in part, be related to poor visualization of the boundaries of stereotactic targets on structural imaging ( ). To address this limitation, specific high-field (3-T) magnetic resonance imaging (MRI) sequences were developed to improve visualization of subcortical nuclei. A majority of the centers are now using T2 fast-spin echo images or proton density weighted images for targeting ( ). Susceptibility weighted imaging (SWI) is also being used for STN targeting ( ). Several other sequences have also been developed and successfully used for surgery for movement disorders ( ). Improved visualization has allowed us to directly target subcortical nuclei for DBS surgery ( ). It is important to understand that direct targeting has limitations due to imaging artifacts; analyzed the correspondence between the STN borders in 196 microelectrode recording (MER) tracks and their prediction with 1.5-T SWI, 1.5-T T2-weighted, and 3-T T2-weighted MRI. The authors found that SWI was consistently overestimating the location of the lateral part of the STN. Although the imaging resolution will continue to improve with high magnetic field scanners (e.g., 7-T) ( ), the increased probability of susceptibility artifacts and geometric distortions needs to be addressed before implementation of this technology for surgical targeting (e.g., a recent study reported that 7-T imaging erroneously detected the dorsal STN border more dorsally ).
In parallel with increased availability and resolution of imaging and the advent of intraoperative three-dimensional imaging modalities ( ), there is increasing interest in performing pure image-guided electrode insertion. A completely MRI-compatible platform for DBS surgery has been developed ( ). published the first reports with this system in six pediatric patients with dystonia with submillimeter accuracy in targeting without significantly increasing the operative times. The outcomes in experienced centers are encouraging ( ) and comparable to those of the MER-based targeting approach ( ). The possibility of the patient being completely asleep for surgery may improve patient comfort and satisfaction ( ). The initial cost implications are also favorable for asleep DBS ( ). Interestingly in comparative studies in ET, the clinical outcomes were also comparable and the accuracy of electrode implantation was better with asleep DBS ( ). A prospective comparison between these techniques is still ongoing ( ).
Substrates of DBS Efficacy and the Origin of Connectivity-Based Targeting
With the recognition of white matter tracts mediating the efficacy of DBS ( ), there is a move toward integrating tractography for personalized stereotactic targeting ( ). Diffusion tensor imaging (DTI) is the modality of choice to analyze the structural connectivity in health and disease states ( ). The challenges associated with susceptibility, motion, and chemical shift artifacts have been reasonably solved with the use of methods for reliable acquisition and robust preprocessing algorithms ( ). A tractography-based approach was recently reported for VIM targeting for tremor surgery ( ) ( Fig. 34.1 ). This approach defines the borders of VIM in addition to its structural connectivity with the motor cortex and cerebellum. The short-term outcomes were encouraging, and the imaging findings were well corroborated with intraoperative physiology ( ). Similar tract-substrates for clinical efficacy are being investigated with STN DBS (e.g., the white matter pathways linking the superior frontal gyrus and the thalamus were associated with favorable clinical outcomes in a recent report ). Initial results from tractography-aided targeting during DBS for depression, Tourette syndrome, and other psychiatric disorders are also promising ( ).

The quest for identification of white matter tracts critical for DBS efficacy has also yielded novel targets for stimulation (e.g., posterior subthalamic zone is a novel target for tremor surgery ). This target has been used extensively for lesioning, but the experience with DBS is limited ( ). Since the afferent cerebellar input can be effectively stimulated at this location, this target may be more efficacious for symptoms control and avoiding long-term tolerance to stimulation ( ). Although some studies have favored one over the other ( ), a randomized comparison between conventional VIM target and posterior subthalamic zone is ongoing ( ). A similar discovery involves the medial forebrain bundle as a therapeutic target for mood disorders. The dysfunction within the reward network is postulated to underlie the pathogenesis of depression ( ). The medial forebrain bundle connects the major nodes (nucleus accumbens, ventral tegmental area, amygdala, and hypothalamus) within this network. A pilot study targeting the bilateral superolateral branch of the medial forebrain bundle included seven patients with MDD ( ). Six patients were classified as responders after a follow-up of at least 3 months, and this trial is actively recruiting a larger number of patients ( ).
Stimulation Titration For Modulation of Dysfunctional Networks With DBS
The initial recognition of dysfunction in the limbic network underlying the pathogenesis of temporal lobe epilepsy ( ) eventually led to the current understanding that pathologic interaction between different “nodes” in brain networks underlies neurologic and psychiatric disorders; for example, in PD, pathologic β oscillations (10–20 Hz) predominate in the motor network during clinical symptoms ( ). A variety of techniques have broadened our understanding of the architecture ( ) and dynamics ( ) of these networks. This framework is now being applied for psychiatric disorders ( ). An abnormality in the default mode network, a subset of cortical areas implicated in self-referential functions, characterizes patients with depression ( ). Similarly, dysfunction in the corticostriatothalamocortical network is implicated in the pathogenesis of OCD ( ).
DBS modulates the activity of functionally connected brain networks mainly via axonal stimulation and alteration in local field potentials (LFPs) ( ). The net stimulation effect on these networks can reestablish the physiologic connectivity, resulting in clinical improvement ( ). DBS has also provided a unique opportunity to better understand this dysfunction and the effects of their disruption ( ), e.g., in a combined magnetoencephalography and STN LFP recordings study, discovered increased coherence within the “frontal network” after l -dopa administration, and this was associated with improved planning of motor execution. It is conceivable that future trials will include connectivity-based markers to stratify patients and use these as predictors of clinical response ( ). Capturing this heterogeneity beyond the clinical phenotypes will eventually allow us to develop a tailored approach for DBS candidacy ( ).
Closed-Loop Stimulation
The conventional stimulation paradigms deliver a constant electrical energy to the brain tissue. Continuous stimulation may have implications for clinical efficacy (e.g., High-frequency STN DBS during medication “on” state may slightly worsen bradykinesia ( )) and device life (e.g., higher energy consumption). In epilepsy continuous stimulation irrespective of seizure onset may excessively drain the battery ( ). Incorporating markers of real-time network state to “close the loop” for stimulation may address some of these concerns (e.g., one strategy may involve simultaneous measurement of the LFPs from either the stimulation target or other downstream nodes ). The most successful application of this type of closed-loop stimulation involves hippocampal and cortical stimulation in patients with refractory epilepsy ( ). Novel algorithms for seizure prediction are being investigated for closed-loop stimulation in other stimulation targets in epilepsy ( ), and their clinical efficacy remains to be investigated ( ).
The implementation of such a recording system within the pulse generator of the DBS device (“Activa PC + S” device; Medtronic Inc.) is one such step in this direction ( ). Using an LFP-based feedback in an “adaptive” device, demonstrated greater motor improvement compared with chronic stimulation with fixed settings and a 56% reduction in stimulation time. The feedback for stimulation may also come from the objective measurements of the network output. recently used an accelerometer-based feedback in patients with VIM DBS for ET. The application of high-frequency burst stimulation time-locked with the “optimal phase” of tremor (the phase that was most likely to reduce tremor in one patient) was more effective than constant stimulation and required less energy.
In a separate line of investigation, the feedback for closing the stimulation loop may also come from the measurement of local neurotransmitters (e.g., measurement of local dopamine using real-time microdialysis ). The low temporal resolution of microdialysis remains a challenge in successful translation of this approach ( ). Recently, proposed a novel method for the measurement of steady-state levels of dopamine, glutamate, and GABA while decreasing lag time to 40 min. Further studies are needed to understand the clinical potentials for this approach outside of research.
Directional Electrodes
It is well known that the accuracy of electrode placement is paramount for achieving good outcomes, and implantation errors of few millimeters may adversely affect therapeutic efficacy ( ). For example, if the electrode is implanted close to the lateral STN border, the “therapeutic window” may be limited due to lower threshold for motor side effects from the stimulation of internal capsule. Although accuracy of implantation may account for some of these instances ( ), the poor predictive value of the intraoperative testing for postoperative thresholds may also be an important factor ( ). To overcome these limitation, “steerable” or “directional” electrodes designs were engineered. These electrodes have the potential for stimulating a “preferential face” of the stimulating contacts, potentially exciting different neuronal components, in comparison to the cylindrical DBS lead, a segmented lead allowed for avoiding the potential side effects of paresthesias and contractions ( ) ( Fig. 34.2 ). Similar results have been reported with other leads: directSTIM lead (Aleva Neurotherapeutics Inc.) ( ), directional lead from Vercise lead (Boston Scientific Inc.) ( ), and “SureStim” lead (Sapiens Inc.) ( ). Stimulation titration can be challenging with increasing number of contacts. Commercial solutions have been developed for efficient testing of efficacy of side effects ( ) with encouraging initial results ( ). Prediction of volume of tissue activation by DBS may be a promising approach for stimulation titration ( ). More recent models include a realistic estimation based on patient tissue diffusivity characteristics ( ) and side effects profile ( ) to accurately understand the spatial relationship with known white matter pathways. This approach will be particularly suited for psychiatric disorders, where the final effects of a settings change may not be objectively measurable ( ).

Novel Stimulation Parameters
In situations with difficult stimulation programing, interleaved stimulation (Medtronic Inc.) allows for independent and alternate stimulation of two contacts using different voltages and pulse widths. This allows for stimulation of anatomically proximal regions with different stimulation amplitudes and shaping of the electric field to avoid side effects. This approach has been studied for both STN ( ) and GPi DBS ( ). Although useful, interleaved stimulation may increase the energy requirements and therefore increase the battery drain. Constant current stimulation (Boston Scientific Inc.), using various amplitudes, frequencies, and pulse widths at different electrodes, is another innovative approach for avoiding side effects while reducing the energy requirements ( ). Clinical reports of STN DBS on the use of this device are encouraging ( ). Theta burst stimulation was initially investigated for transcranial magnetic stimulation, simulating the physiologic pattern of hippocampal neuronal firing ( ). It has also been used for cortical stimulation ( ), and its appeal for DBS derives from a potential to decrease energy consumption compared with the chronic “tonic” stimulation. In a blinded assessment, reported a stimulation-locked (200 Hz in 100-ms trains, 5 trains/s, 100 μs, 7 mA) marked improvement in the immediate and delayed cognitive performance (Medical College of Georgia Complex Figure Test) after fornix and hippocampal stimulation in patients with drug-resistant epilepsy. Future investigations with larger sample sizes are needed to further explore the clinical utility of this stimulation paradigm.
Conclusions
The clinical effects of DBS are often immediate and dramatic. Several technique and technological advances have the promise of reducing the variability of outcomes. With the recognition of white matter simulation underlying DBS therapeutic efficacy, tractography-based targeting methods are going to play a critical role in targeting networks of interest. In parallel, incorporating real-time feedback of network modulation will increase the stimulation efficiency and potentially improve the overall clinical outcomes.
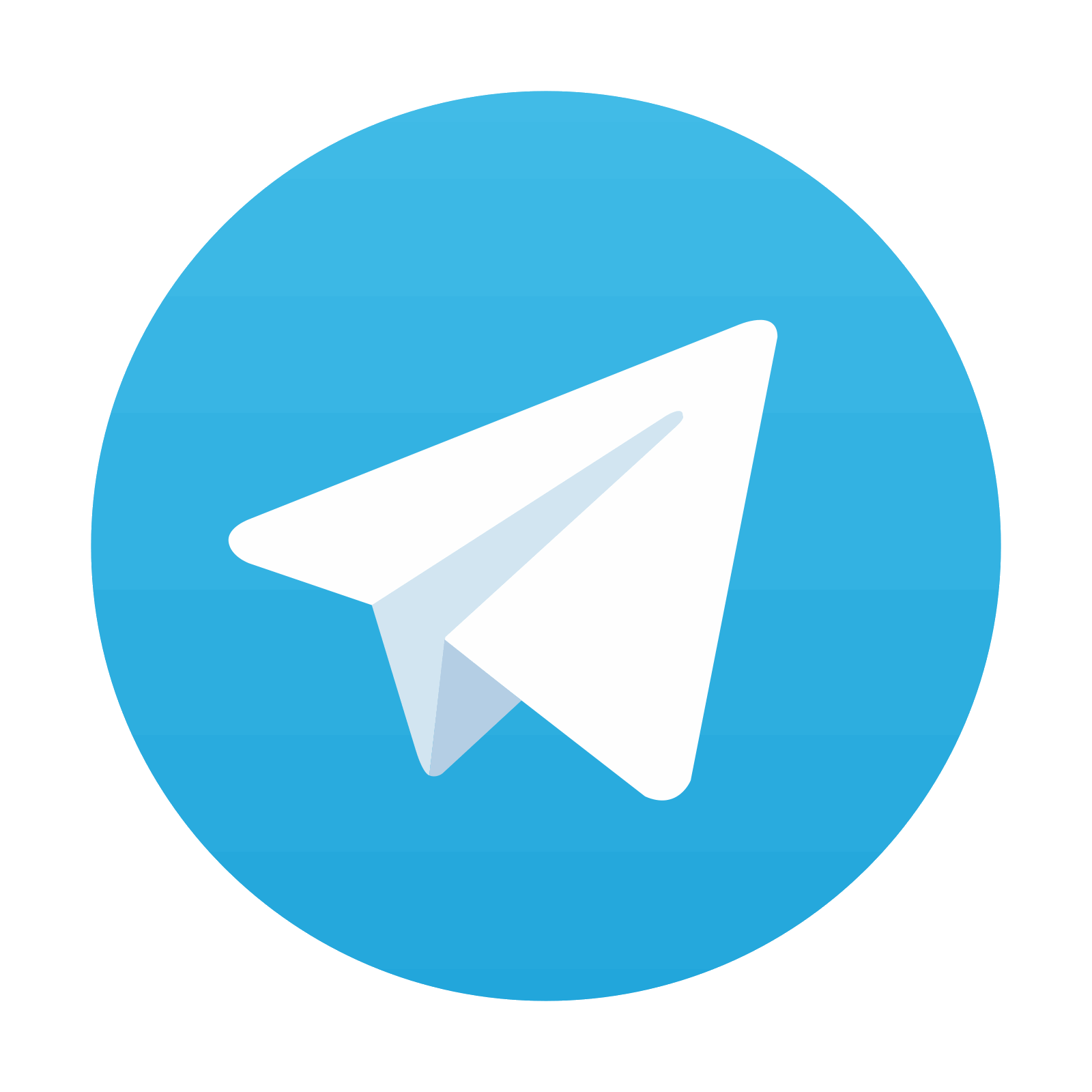
Stay updated, free articles. Join our Telegram channel
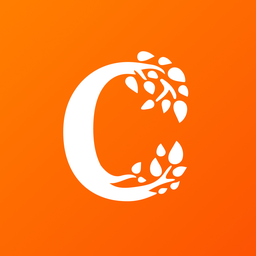
Full access? Get Clinical Tree
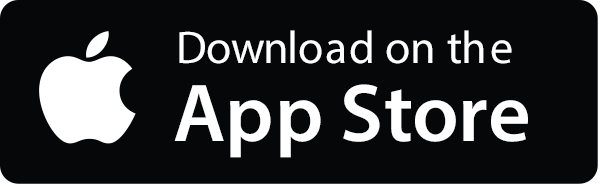
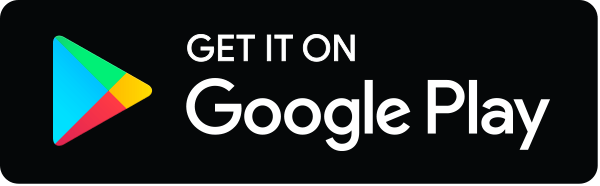