Abstract
This overview introduces the methodology, history, mechanisms, and applications of transcranial Direct Current Stimulation (tDCS). This includes tracing the historical origins of tDCS along related transcranial Electrical Stimulation (tES) techniques such as Electrosleep, Cranial Electrotherapy Stimulation, and Limoge Current. tDCS terminology and tDCS dosaging is defined. How tDCS is customized to given applications is explained (the origins of tDCS specificity) and includes anatomical, activity-selective, and input-bias mechanisms. Preparation methods for tDCS are reviewed that include selecting and preparing electrodes and contact medium, selecting and preparing electrode placement, common montages, and the use of computational models to optimize dose. We review safety considerations for tDCS and important efficacy trials.
Keywords
Brain stimulation, tDCS, tES, Transcranial Alternating Current Stimulation (tACS), Transcranial Direct Current Stimulation
Outline
Introduction 1589
Historical Background and Other Transcranial Electrical Stimulation Approaches 1590
Developments From Electrosleep to Cranial Electrotherapy Stimulation 1590
Developments From Electroanesthesia to Limoge Current and Other Related Methods 1592
Development of Direct Current Stimulation 1592
Transcranial Direct Current Stimulation Customization 1593
Transcranial Direct Current Stimulation Terminology 1593
Transcranial Electrical Stimulation and Transcrianial Direct Current Stimulation “Dosage” 1594
Origins of Specificity During Transcranial Direct Current Stimulation: Anatomical, Activity-Selective, and Input-Bias Mechanisms 1596
Anatomical Transcranial Direct Current Stimulation Specificity and the “Sliding-Scale” Model 1597
Activity-Selectivity and Task-Specific Modulation 1598
Input-Selectivity and Bias 1598
Preparation for Transcranial Direct Current Stimulation Stimulation 1599
Selecting and Preparing Electrodes and Contact Medium 1599
Selecting and Preparing Electrode Placement 1599
Computational Models of Transcranial Direct Current Stimulation for Electrode Placement 1601
Common Montages 1601
Methods for the Generation and Use of Computational Forward Models of Transcranial Direct Current Stimulation 1601
Safety Considerations for Transcranial Direct Current Stimulation 1602
Transcranial Direct Current Stimulation Safety Data From Human Trials and Models 1603
Efficacy 1604
References 1605
Introduction
Since transcranial Direct Current Stimulation (tDCS) is an emerging technology, it is useful to introduce it in the context of other established neuromodulation techniques. Electrotherapy approaches using implanted electrical stimulators, such as Deep Brain Stimulation (DBS) ( ), and Vagus Nerve Stimulation (VNS) ( ), are increasingly being used to treat neurological (e.g., refractory movement disorders) and psychiatric disorders (i.e., severe refractory mood and anxiety disorders). However, these approaches inherently require surgery and are thus associated with several manifest limitations related to cost and risk, and do not provide the adaptive flexibility of noninvasive approaches ( ). Noninvasive approaches offer the promise of noninvasive and flexible interventions. Among the most established noninvasive electrotherapy approaches, Electroconvulsive Therapy (ECT) and repetitive Transcranial Magnetic Stimulation (rTMS) are US Food and Drug Administration (FDA) approved but are associated with costs (e.g., in-office treatments and anesthesia) and side effects (e.g., pain and memory loss). tDCS is an emerging, noninvasive technique that offers the promise of electrotherapy with minimal cost and side effects.
tDCS is a simple and customizable technique that is applied to a wide range of clinical and cognitive neuroscience applications ( ). It is a promising tool in cognitive neuroscience and neuropsychiatric therapy with interventions rationalized and based on current to targeted brain regions to modulate excitability ( ) and plasticity ( ).
An ideal electrotherapy treatment would combine ease of administration (ultimately, self-administered at home), effective outcomes through plastic brain changes (not requiring chronic stimulation), low-cost, robust safety (noninvasive, with no major risks), and thus minimal contraindications. From this perspective, tDCS is a highly promising electrotherapy approach ( ). However, there still remain questions about the clinical efficacy of tDCS.
Historical Background and Other Transcranial Electrical Stimulation Approaches
The emergence of tDCS is important to understand in the context of over a century of electrical stimulation history. Such a review also helps to explain and disambiguate terminology used to describe related techniques. Transcranial Electrical Stimulation (tES) encompasses all forms of research and clinical application of noninvasive electrical currents to the brain using electrode/s (at least one) on the head. The dose of tES is defined by the electrode montage and the stimulation waveform applied to the electrode ( ). There has been a resurgence of interest since 2000, but tES developed incrementally over a century. Historically, there are “streams” of linked categories of tES ( Fig. 135.1 ): (1) Cranial Electrical Stimulation (CES) descended from Electrosleep (ES) through Cranial Electrostimulation Therapy (CET), Transcerebral Electrotherapy (TCET), and Neuroelectric Therapy (NET); (2) Electroanesthesia (EA) went through several periods of waning interest and resurgence when new waveform variations were proposed including Transcutaneous Cranial Electrical Stimulation (TCES), Limoge, and Interferential Stimulation; (3) Polarizing or Direct Current Stimulation includes recent tDCS, Transcranial Micropolarization, High-Definition transcranial Direct Current Stimulation (HD-tDCS) and Galvanic Vestibular Stimulation (GVS); (4) ECT, initially called Electroshock Therapy, evolved in technique and dose, such as Focal Electrically Administered Seizure Therapy (FEAST); and (5) “Contemporary” approaches that have been explored intensely over the last decade, such as transcranial Alternating Current Stimulation (tACS), transcranial Sinusoidal Direct Current Stimulation (tSDCS), and transcranial Random Noise Stimulation (tRNS). Though analogues to these contemporary approaches can be identified in earlier literature, contemporary methods contain dose features that motivate us to consider them novel as a category. Contemporary approaches, along with the rediscovery of tDCS, to some extent, reflect a “reboot” of interest in tES approach with modern technology and clinical research approaches ( ).

Several “streams” of tES began around 1900s ( ), and included ES and EA—which are described in the next two sections.
Developments From Electrosleep to Cranial Electrotherapy Stimulation
ES is the name for which the brain was stimulated to induce a sleep-like state in the subject. The first studies on ES were initiated in 1902 ( ); however, the first clinical report of ES was published 12 years later by Robinovitch ( ). New approaches, such as changing electrode position from covering the eyes to locations around the eyes, presumably to “reduce optic nerve irritation” ( ) were developed, mostly in Europe. ES dose waveform was typically pulsed at 30–100 Hz, but at least one (unsuccessful) case of use of DC current was documented ( ). In a symposium, it was reasoned that ES does not actually induce sleep, rather it is an indirect side effect of the relaxing effects of stimulation. Therefore, the name of ES was changed to CET ( ). This was the first of several changes of the name of ES over the next few decades, often with notable changes in dose. In 1969, TCET was proposed as another alternative name. However, such devices were renamed as CES ( ).
A notable device, produced after the name change to CET, was the Neurotone 101 , which was based on a Russian ES device brought to the United States. Although the Neurotone 101 is no longer in production, it was the first device to be approved by the FDA as a CES device ( ) and all subsequent CES devices approved by the FDA, such as the Alpha-Stim, the Oasis Pro, and the Fisher–Wallace Stimulator ( Fig. 135.2 ), were through a 510k process claiming equivalency, either direct or descendent, to the Neurotone 101.

Modern CES is thus a historical descendent of ES, even as dose and indications have continuously evolved. The FDA sanctioned CES in 1978 through a “grandfather clause” and have not, since then, advanced a regulatory consensus, even as more variants were being developed. CES, in this modern form, excludes DC waveforms, so it is distinct from tDCS, but its historical predicates include DC components.
Developments From Electroanesthesia to Limoge Current and Other Related Methods
EA, in short, was intended to induce anesthesia in the subject using high frequency stimulation so that chemicals did not have to be used, presurgery. EA studies started in 1903, but were first known as Electronarcosis (EN) ( ). Russian scientists used the term “EA” to describe local anesthesia, while “EN” described general anesthesia ( ). However, EA stopped being referred to as local, as applied to the periphery, and began to be known as general anesthesia, as applied to the brain.
Research into EA dosage continued and the term TCES was adopted around 1960–63. Even though the term TCES was not adopted until the early 1960s, similar protocols were used, as early as 1902 by Leduc ( ). In 1951, Denier proposed that high frequency trains of 90 kHz could be used to avoid muscular contraction ( ). Three years later, claimed that alternating currents (AC) at 700 Hz should be applied, but this was abandoned in 1958 due to cardiovascular complications ( ). In 1957, investigators in the Soviet Union attempted to add a Direct Current (DC) component to Leduc’s currents but, as claimed by an American scientist, Robert Smith, it resulted in a collection of undesirable side effects ( ). In 1964, a study claimed that pulsating currents are more effective than DC for the induction of EA ( ). Another study suggested that the use of pure DC for EA required high intensities of approximately 40 mA ( ). EA, and its derivative technologies, are not explicitly integrated into contemporary neuromodulation research and treatment, but represent important historical precedents that include cases of testing of DC waveforms.
Development of Direct Current Stimulation
DCS has been used intermittently as a component in both ES and EA. In 1957, a DC bias was added to ES, which is traditionally applied using only AC or Pulse Current (PC). The advent of TCES, around 1960–63, in the third resurgence of EA research, also incorporated a DC bias. In 1969, pure direct current stimulation was investigated for inducing anesthesia ( ). However, it was not until 1964 that preliminary studies, heralding modern tDCS, were published ( ).
In 1964, Redfearn and Lippold investigated Polarizing Current for the treatment of neuropsychiatric diseases ( ). Their use of prolonged (minutes) of stimulation was motivated by animal studies showing that prolonged DCS could produce lasting changes in excitability ( ). While further open, pilot studies and clinical observations suggested efficacy ( ), a following negative, controlled trial ( ) seems to have halted investigation (at least as published in Western journals) for several decades.
The neurophysiological basis of neuromodulation using short-duration tDCS was investigated by Priori et al. in . Shortly after, Nitsche and Paulus established that prolonged (minutes) tDCS could produce lasting and polarity-specific changes in cortical excitability ( ). This was followed by pilot clinical studies ( ) for indications spanning depression ( ), pain ( ), epilepsy ( ), and a broad range of neuropsychiatric disorders ( ). tDCS is further explored for rehabilitation after stroke ( ). Moreover, due to the perceived safety of tDCS, it was initially validated for neurophysiological changes in healthy subjects and continues to be investigated in healthy individuals for changes in behavior and cognitive performance ( ).
In 2007, HD-tDCS was proposed as a focalized form of tDCS ( ). HD-tDCS electrodes were designed for increased charge-passage capacity through a smaller contact area ( ), arranged in arrays that can be optimized per indication ( ). HD-tDCS montages tested have included the 4 × 1 configuration ( ) as well as individually optimized arrays ( ). The focalization of current with HD-tDCS is an improvement upon tDCS, where previously a broad area would be stimulated, and now specific targets can be stimulated. Some of the devices that have been used are the Schneider (tDCS), Soterix Medical 1 × 1 (tDCS), and the Soterix Medical 4 × 1 (HD-tDCS) ( Fig. 135.2 ).
Transcranial Micropolarization is a technique investigated in Russia which is a modified version of tDCS that uses small electrodes instead of pads and currents up to 1 mA, that are claimed to be “weak” ( ). GVS has been extensively tested for effects on ocular and postural movement ( ). Alongside GVS, Caloric Vestibular Stimulation (CVS) is under investigation due to similar areas being targeted by stimulation. However, CVS does not utilize electricity; rather, it uses irrigation of the ear canal using cold or warm water ( ).
Transcranial Direct Current Stimulation Customization
Transcranial Direct Current Stimulation Terminology
To accurately describe tDCS and, more broadly, tES, electrical therapy “dosage,” it is important to have a basic understanding of the terminology (system of metrics) that is used to define and differentiate the various practical aspects of tES. It is useful to clarify how electrical therapy terms are used (sometimes inconsistently) in the literature.
- •
Stating that a protocol employs “DC” stimulation indicates that the stimulation remains at peak intensities for the duration of the exposure.
- •
tDCS/DCS is current controlled, meaning the voltage is varied to maintain a fixed current, typically under 20 V ( ), though much of this voltage (especially, any time-dependent component) may reflect the electrode and skin impedance.
- •
“Monophasic” stimulation indicates that, for the entire exposure, though the intensity may vary, only one polarity is applied (current is passed only in one direction).
- •
“Biphasic” and “AC” stimulation indicate that, at some point in the course of an exposure, the polarity is reversed, which is not consistent with tDCS.
In the brain, electrical stimulation, anode, and cathode terminology should always be used consistently for indicating the electrode where positive current is entering the body (anode) and the electrode where positive current is exiting the body (cathode) ( ). For tDCS/DCS, using two electrodes, there is one fixed anode and one fixed cathode, with the anode at a positive voltage, relative to the cathode. In clinical and animal studies, anodal stimulation or cathodal stimulation would indicate that a cortical region of interest (target) was nearer the anode or the cathode, respectively. It is important to recognize stimulation always includes an anode and cathode.
In classic animal literature, the terms “surface positive” and “surface negative” correspond to an anode or cathode electrode, respectively, placed on the surface of the cortex, with the other electrode often placed on the neck or body. Considering the cortical surface, inward current and outward current are typically expected under the anode and cathode, respectively (though cortical anatomy may produce deviations). For electric field, the direction also needs to be specified ( ). Datta et al. adopted the convention that an inward current will produce a positive electric field, measured from outside pointed in, while an outward current will produce a negative electric field, measured ( ); unless otherwise stated, it is implied that the current and electric fields are normal/orthogonal to the cortical surface, rather than tangential/parallel ( ). Current density, as used in the literature, indicates the average current density (in ampere per square meter or A/m 2 ) at the electrode, calculated by taking the applied current to a given electrode and dividing by electrode area. Average current density is not necessarily indicative of peak current density at the electrode (which may be concentrated at edges or spots ( ); or in the brain (which depends on many other factors, namely head anatomy; )). Stimulation charge (in coulombs, C) is determined by multiplying current by duration. Stimulation charge density (A × t/m 2 = C/m 2 ) is charge divided by electrode area, and is also an average metric. Stimulation power (in W W = V × A) is voltage multiplied by current. Stimulation energy (in joules, J = V × A × t) is power multiplied by duration. For any tDCS session, the “summary” metrics are a single number, or a single number per electrode, and determined fully and only by dose ( ). Electric field (in V/m) is current density multiplied by local tissue resistivity. The peak current density or electric field represents the maximum value at any point in space, which can be further restricted by head region such as peak current density in the brain or skin. The electric field predicts neuronal activation threshold more meaningfully than current density, but it is very sensitive to assumptions on local tissue resistivity. It is not established whether injury is linked to neuronal activation (e.g., excitotoxic). The tissue properties are not time dependent, but can be combined with time in new metrics ( ).
Transcranial Electrical Stimulation and Transcrianial Direct Current Stimulation “Dosage”
tES encompasses all research and clinical technology to modulate brain function by passing current through at least one electrode placed on the scalp. tDCS involves relatively weak (≤2 mA) DCS for several minutes. CES encompasses a range of pulsed protocols (>1 Hz) with intensities nominally <5 mA. ECT involves relatively strong (>0.9 A) pulsed waveforms, sufficient to induce electrographic seizures. tDCS and CES are both subconvulsive. The potential range of electrotherapy stimulation paradigms extends well beyond the limited set currently described by tDCS, CES, and ECT. However, clinical treatment with TCS has been generally restricted to this limited set of stimulation protocols, reflecting both safety concerns and restricting innovation and limitations of common clinical stimulation devices. Even within a specific therapy classification (e.g., tDCS), there are “dosage” variables that will fundamentally affect clinical outcome ( ).
For any tES, including tDCS research or therapeutic application, the electrotherapy paradigms (“dosage”) will be fully described by the following system of six independent metrics. This system is based on clinical experience with factors found to effect outcome as well as in vivo and in vitro animal and human studies on stimulation mechanisms ( ). The tES device consists of a programmable stimulator (metrics 1, 4, 5) connected via leads to electrodes placed on the body (metrics 2, 3). In some stimulation paradigms, multiple stimulation devices may be simultaneously activated, in which case, stimulation parameters (metrics 1–6) may be “mirrored” (e.g., same stimulation protocol but opposite hemispheres) or distinct for each device.
- 1.
Exposure Duration : A single exposure is defined in the context of the outputs of an electrical stimulator being energized (turned from OFF to ON) and then being turned OFF after a user-determined exposure duration. When the stimulation is OFF no current/voltage is being applied to the electrodes/patient. When stimulation is turned ON it is assumed that the remaining metrics defined in 2–5 of this list are fixed for the duration of any given exposure (e.g., electrodes are not moved during an exposure). If exposure duration is adjusted across patients (metric 7), indicate the range.
- 2.
Electrode number, connectivity, and position on body : It is assumed that the default stimulation device has two output wires and each electrode is connected to only one of these two wires. There may be more than two electrodes per stimulation device, such that multiple electrodes are connected to the same output wire. This connectivity and the respective position of every electrode on the surface of the body must be indicated. The position of all scalp electrodes and extracephalic electrodes must be accurately reported. It is not sufficient to indicate only the presumed “active” electrodes. In case of tDCS, each electrode can be designated either an “Anode” or a “Cathode,” which indicates connectivity to the respective stimulator, output terminal.
- 3.
Electrode size/shape, electrode material, and contact/skin conditions : The geometry (shape) and size of every electrode can fundamentally impact therapy outcome ( ). The electrode material should be indicated as well as the use of any specific electrolyte gel and sponge (including salinity/conductivity of sponge fluid; ). The use of conductive solution/gel may change the “effective” area of the electrode. The preparation of the skin should also be described (e.g., no preparation, cleaning skin with alcohol, or abrasive).
- 4.
Stimulation peak intensity (stimulation peak amplitude) : Indicate whether the stimulation device is “current controlled” or “voltage controlled.” Indicate the peak intensity of the stimulation as the maximum amplitude of current (for current controlled) or voltage (for voltage controlled), applied to the electrodes during the entire exposure. For the case of tDCS, peak intensity is nominally the intensity of the DCS. If peak intensity is adjusted across patients (metric 7), indicate the range.
- 5.
Waveform : The stimulation waveform fully describes the output of the stimulation device during the entire exposure duration (from turning stimulation ON to OFF, including any designed or undesired transients). No aspect of the waveform can be ignored or omitted, including initial transients. The waveform describes how the current (for current controlled) or voltage (for voltage controlled) applied to the electrodes changes over time, never exceeding the peak intensity. Often, the waveform can be described using simple mathematical functions such as ramps, sinusoids, or pulses. It is necessary that in the description of the waveform(s), any change in polarity should be indicated. For the case of tDCS, the waveform does not change polarity (in a given exposure) and remains DC for the majority of the exposure duration; however, the initiation and termination of stimulation may be associated with other waveform components (e.g., on/off ramps).
- 6.
Exposure number and interval : These parameters fully describe the stimulation paradigm (“dose”) used for a single exposure. For multiple exposures, it is also necessary to describe the total number and interval between exposures. For multiple exposures, the stimulation metrics (1–5) for each exposure may change. Since tDCS may have prolonged effects on neuronal excitability ( ) and mood, repeated exposures may interact in a complex fashion. This is of particular clinical significance, as repeated exposure of the same polarity may simplistically lead to cumulative effects, while alternating polarity may potentially interfere with net effects ( ).
Each commercial clinical stimulator produces a limited range/combination of intensities and waveforms, and is often recommended with specific electrode configurations. Theoretically, if the factors are all controlled for, the specific manufacturer/model of stimulation and electrodes will not affect therapy outcome (no more than if two companies produced chemically identical drugs). However, therapeutic devices may not perform ideally (e.g., deliver the peak intensity indicated), in which case, reporting the device used and device settings will allow for post hoc correction of unintended device performance ( ).
Just as with pharmaceutical clinical trials, the methodologies and controls used in an electrical therapy trial (including but not limited to dose) will determine how each specific trial is interpreted. Particularly in psychiatric trials, special consideration must be given for potential placebo effects, related to device operation (e.g., beeping) and electricity sensation. As with pharmaceutical trials, a dose-response study may be indicated under certain circumstances; each electrical “dosage” metric (1–6) may be changed independently. However, as in a fixed-dose pharmaceutical study, the “electrotherapy dosage” (metrics 1–6) once standardized, needs to be administered reproducibly for any meaningful conclusions as to efficacy and safety, to be drawn.
There have been considerations in the clinical literature to summarize the dosage of a complete stimulation paradigm, using a single “reduced” metric. Examples of reduced metrics include peak electrode current density (the peak current divided by the area of a selected electrode) or charge-per-phase (the average current in a specific pulse times the duration of the pulse). Working with limited, stimulation, paradigm constraints (e.g., DCS through large scalp electrodes), these single metrics provide general guidelines in normalizing the effects of therapy across subjects and studies (e.g., maintain current density across electrode sizes). However, these reduced metrics do not necessarily serve as absolute normalizing factors for efficacy/safety and simply reporting these metrics does not fully describe the stimulation paradigm; that is, it is not possible to determine/reproduce the stimulation paradigm given only a reduced metric ( ).
From the perspective of normalizing stimulation across patients (including efficacy and safety aspects), current-as opposed to voltage-controlled stimulation is preferred. Although voltage-controlled stimulation remains “grandfathered” into some stimulation technologies, with voltage control the electric field induced intracranially (which ultimately determines therapy effects) is “not controlled” across patients ( ), and will vary in an unpredictable fashion, depending on electrode size and material and skin properties ( ). Main features distinguishing modern tDCS from other stimulation techniques are the weak and diffuse electric fields, induced in the brain ( Fig. 135.3 ).

The typical dosage during tDCS (1 mA stimulation intensity) produces ≤0.2 V/m electric field in the brain ( ). Animal experiments have shown that such weak fields change the membrane potential by ∼0.2 mV ( ). Neurons, at rest, typically require 15 mV depolarization to reach firing threshold. How can such weak electric fields produce significant changes in brain function?
In addition to being weak, the induced electric field in the brain is highly diffuse when using conventional (non-HD) tDCS montages ( ). Current flows from the anode to cathode, while generating equivalent electric field magnitudes in both the target brain region and adjacent brain regions ( Fig. 135.4 ). These areas may be part of the functional network that is being targeted. Additionally, brain regions that are not being targeted are also exposed to the electric field. This raises a question of the specificity of electrical stimulation. How can a diffuse electric field, which simultaneously polarizes neurons across the brain, have precise effects on brain functions?

Origins of Specificity During Transcranial Direct Current Stimulation: Anatomical, Activity-Selective, and Input-Bias Mechanisms
tDCS is investigated for a broad range of neuropsychiatric indications, used for various rehabilitation applications, and used to modulate cognitive performance in diverse tasks. Specificity of tDCS refers broadly to the ability of tDCS to produce precise, as opposed to diffuse, changes in brain function. Practically, specificity of tDCS implies application-specific customization of protocols to maximize desired outcomes and minimize undesired effects. Especially given the simplicity of tDCS and the complexity of brain function, understanding the mechanisms leading to specificity is fundamental to the rational advancement of tDCS.
Bikson et al. grouped the origins of specificity based on anatomical and functional factors ( ). Anatomical specificity derives from guiding current to targeted brain structures. Functional specificity may derive from active, neuronal networks that are preferentially modulated by tDCS. Rational advancement of tDCS may require leveraging all forms of specificity.
Anatomical Transcranial Direct Current Stimulation Specificity and the “Sliding-Scale” Model
Anatomical specificity refers to the preferential neuromodulation of targeted brain regions by delivering stimulation current to the targeted area. The number, location, and size of anatomical targets are application specific. For example, the targeted, brain region may be a specific cortical area, implicated in a task or pathology. Anatomical specificity is achieved only through the control of tDCS electrode dose (defined as electrode montage and current) to guide current to specific brain regions ( ). However, applied without consideration for functional specificity, anatomical specificity is technically and conceptually limited.
Both computational models of current flow in the brain and imaging studies indicate that conventional tDCS methodology using two large sponge pads (5 × 5 cm) produce dispersed current through much of the cortex ( ) and even deep brain structures ( ). It is important to distinguish between well conducted studies that demonstrate dose-specific (e.g., electrode position) outcomes ( ), from implications that current flow is limited to one brain target. These studies also typically leverage other forms of functional targeting.
Technology for HD-tDCS using arrays of electrodes allows categorical increases in anatomical targeting by increasing the focus of current flow ( ), but even so, any brain region is evidently involved in multiple tasks. Which presents the inherent conceptual challenge when relying exclusively on anatomical specificity: How can passing DC current through a multitasking, complex, brain region produce specific functional changes?
In the absence of further sophistication, the goals of tDCS are often described as increasing excitability (near the anode) or decreasing excitability (near the cathode) of the target brain region, with brain function and disease thus reduced to a “sliding-scale” of excitability, to be adjusted by stimulation (cf., ). For example, under the sliding-scale concept “anodal tDCS” can enhance the performance of a cognitive task by exciting an implicated brain region. Similarly, anodal tDCS is intended to increase left, prefrontal cortex activity in depression and enhance rehabilitation around lesions, after stroke. Following early evaluations of transcranial/transcortical polarization in humans and animal models ( ), influential neurophysiological studies of tDCS ( ) established modulation of experimental, evoked potentials (e.g., motor-evoked potential responses to Transcranial Magnetic Stimulation (TMS)). There is significant extrapolation from these experimental findings to behavior and cognition (TMS evoked responses may provide poor evidence for effects on behavior ). Moreover, even the direction of this basic modulation of experimentally, evoked potentials is highly sensitive to both tDCS dose intensity ( ), direction ( ), and dependent on brain state ( ). Animal studies that show anodal/cathodal DCS producing somatic depolarization/hyperpolarizing ( ), and increase/decrease in firing rate ( ), are cited to support a sliding-scale concept; however, global changes in firing rate across a brain region implies a nonspecific effect ( ). In summary, it is reasonable to conclude from neurophysiologic studies that tDCS can produce dose-specific changes in brain functions ( ) that can, with careful extrapolation, serve as a basis for behavioral interventions ( ). However, relying solely on anatomical specificity by guiding current to specific brain regions (and so the “sliding-scale” rationale) remains limited by the complex and divergent functions of any brain region.
Further sophistication in anatomical targeting follows from considering tangential as well as radial inward/outward currents ( ). Indeed, the assumption of inward (“excitatory”) and outward (“inhibitory”) current under the anode and cathode, respectively, may be a further oversimplification. Electrophysiological studies, in animal models of DCS, suggest differential processing of afferent information ( ) and that polarity-specific effects invert due to neuronal morphology ( ).
Activity-Selectivity and Task-Specific Modulation
Activity-selectivity refers to tDCS preferentially modulating a neuronal network that is already activated, while not modulating separate, neuronal networks that are inactive. The active, neuronal network may be activated for a host of reasons described. The active and inactive networks can in fact overlap in space (e.g., in the same cortical column) such that activity-selectivity does not require physical separation, in contrast to anatomical specificity. Therefore, we refer to activity-selectivity as a form of functional specificity. The active network may represent a subset of neurons and/or a subset of connections (synapses). Because tDCS produces low-intensity, electric fields in the brain, “subthreshold” neuromodulation may reflect changes in ongoing processes, in contrast to suprathreshold driven firing by TMS. Activity-selectivity thus assumes there is some feature of the active network that makes it preferentially sensitive to modulation by tDCS when compared to other inactive networks. We consider two neurophysiological substrates for this preferred sensitivity: ongoing activity-selectivity and input-selectivity.
Activity-selectivity is based on the assumption that tDCS will preferentially modulate specific forms of ongoing activity. For example, at a cellular level, DCS may enhance plasticity in a given synaptic pathway while stimulated at a preferential frequency (0.1 Hz in ( )) or consolidate a specific pattern of activity, presented during DCS ( ). DCS may preferentially modulate the level of potentiation in the activated pathway ( ). It may facilitate long-term potentiation through membrane polarization and removal of Mg+ block ( ), but only those pathways activated during DCS (by a task or experimental stimulation) would benefit from this facilitation. It may be too weak and/or unspecific in isolation to enhance synaptic efficacy, but may boost ongoing (e.g., Hebbian) plasticity, activated by task performance (i.e., modulation of input specific plasticity along an activated synaptic pathway while sparing quiescent synapses). In humans, TES may also preferentially modulate networks with heightened oscillatory activity ( ) or preferentially change the progression of an active network during memory consolidation or synaptic downscaling ( ).
At a behavioral level, specific brain activity is often targeted by training, in conjunction with tDCS, with the goal that this select activity be sensitized to tDCS neuromodulation (and so, it implies that other brain functions, not active in training, may be less so). For example, use-dependent modulation and learning of motor skills is modulated by tDCS ( ). Clinically, tDCS is often applied to enhance the efficacy of rehabilitation or cognitive training ( ), which may further confer functional specificity through activity-selectivity. Clinically, when tDCS is applied to subjects at rest, we can speculate that any functional specificity results from increased sensitivity of pathological network activity to tDCS (e.g., dysfunctional pain or mood regulating networks). It has been speculated that altered network function, associated with brain injury (stroke), may alter the susceptibility to tDCS ( ). Generally, any interaction between brain activity and the efficacy of tDCS modulation ( ) suggests that “tDCS can be highly focal when guided by a behavioral task” ( ).
Although the mechanisms may vary, in any case, functional specificity through activity-selectivity presumes that the enhanced activity of the network makes it preferentially sensitive to modulation by tDCS. Thus, activity-selectivity necessitates an ongoing network process becoming preferentially tuned to influence by DCS when compared to the myriad of other ongoing (background) brain functions.
Input-Selectivity and Bias
A third form of specificity is input-selectivity, which assumes a neuronal network that is predisposed to serve at least two functions or operate in at least two states, such that tDCS can switch the network from one function/state to another (for example, attentional bias in the prefrontal cortex ( )). tDCS would change the state of the system toward a different input bias and thus enhance information processing of a specific stream of information. Input-selectivity may activate endogenous “gating” systems (e.g., gate theory of pain) or bistable, neuronal states, where a nonspecific DC signal is able to “switch” a system between complex functions or modes. In contrast to a sliding-scale hypothesis for a stimulated brain region or activity-selectivity affecting a specific ongoing process, input-selectivity implies that a regional process is enhanced, at the cost of another process, and not that input-selectivity results in a zero-sum effect in regard to lasting cognition or behavior outcomes. However, input-selectivity does emphasize the “cost” of acute stimulation. Input-selectivity is also considered a form of functional specificity since it does not require gross anatomical targeting of current flow. Input-selectivity thus differs conceptually from functional-selectivity, in that it does not presuppose coactivation (e.g., by training), and moreover implies that one process may be enhanced at the cost of enhancing another.
Animal studies that have investigated the modulation of information processing, for example, through synaptic efficacy (as opposed to simply membrane polarization and excitability ( )), have observed that DCS will differentially modulate incoming inputs. We initially showed in hippocampal slice that DCS enhances some and inhibits other afferent inputs ( ), a finding verified ( ) and extended to the cortex ( ). The cellular origins of bias in favor of selective inputs are two-fold. First, although anodal and cathodal tDCS are mistakenly referred to as depolarizing and hyperpolarizing, it is more accurate to describe tDCS as redistributing polarization across the cellular axis (for example, one dendritic branch vs. another ( )). This change in “weights” across the dendrite may provide a cellular substrate to influence the input bias of a network. Second, polarization of afferent axons, itself, appears to exert pathway specific modulation ( ; ).
Preparation for Transcranial Direct Current Stimulation Stimulation
The purpose of electrodes in tDCS is to facilitate delivery of current from the stimulation device to the scalp. Referring to the comprehensive paper by A.J. Woods et al., it is important to prepare electrodes and contact medium, to optimize electrode placement, to adhere to established tDCS protocols, to train the operator, and to use certified devices to prevent tDCS sessions without skin injury ( ).
Selecting and Preparing Electrodes and Contact Medium
The electrode assembly most commonly used for tDCS comprises (1) a metal or conductive rubber electrode, (2) an electrode sponge, and (3) an electrolyte-based contact medium (e.g., saline, gel, or conductive cream) to facilitate delivery of current to the scalp, as well as (4) any materials used to shape these components or otherwise direct current flow (plastic casing, rivets). During tDCS, the metal or conductive rubber electrode is the site of electrochemical reactions ( ) and should not directly contact the skin. Rather, an electrolyte is used as a buffer between the electrode and the skin with sufficient electrolyte volume preventing chemicals formed at the electrode from reaching the skin ( ). The electrolyte can be placed in a sponge encasing the electrode (i.e., saline) or, in the case of electrode cream, placed directly on the electrode surface. However, in the case of saline, oversaturation of the electrode sponge significantly undermines the reproducibility of tDCS application and effects. When sponges are oversaturated, saline is evacuated from the sponge and covers an area of the scalp outside of the surface area, electrode sponge. Rather than delivering current through a specified surface area on the scalp under the electrode (e.g., 5 × 5 cm), the electrode surface area and area of current delivery now encompasses the entire area of the scalp that is covered in saline. This creates an amorphous area of current delivery that is not reproducible within or between subjects. It is important to obtain good contact under, and only under, the electrode with the electrode sufficiently, but not overly saturated. Methods, allowing quantification of saline (e.g., syringes), can assist in achieving a consistent and appropriate amount of contact medium. Whereas, traditional tDCS preparation techniques involves assembly of the electrode components for each session and manual saturation ( Fig. 135.5,A1 ), updated methods use presaturated, assembled sponges with a snap connector. The sponge is saturated and designed for optimal contact.

Consistent with issues introduced by oversaturation of sponges, the shape/size of electrodes/sponges significantly alter the distribution of current that is delivered to the scalp and the brain ( ). At a constant, current intensity level (e.g., 1 mA), increases in electrode size or differences in electrode assembly shape result in differences in the distribution of the current across the surface area of the scalp, resulting in differences in the distribution of current throughout the brain ( ). Thus, it is critical for investigators to consistently report, not only the current intensity applied and the amount of contact medium used, but also the shape and size of the electrode assembly.
Selecting and Preparing Electrode Placement
A critical consideration for tDCS is determining where to place electrodes on the head. Studies monitoring physiological changes following tDCS and computational modeling studies of predicted, current flow demonstrate that the relative location of electrodes results in significant differences in where and how much current is delivered to the brain ( ). For example, demonstrated that relative differences in electrode locations altered whether or not tDCS impacted TMS generated motor-evoked potentials (MEPs). Numerous, modeling studies have demonstrated significant differences between relative locations of electrodes, with results varying from stimulation of the whole brain to more selective stimulation of particular lobes of the brain ( ). further demonstrated that as little as 1 cm of movement in electrode position significantly altered the distribution of predicted current flow in the brain, as well as the intensity of stimulation in specific brain regions. Computational modeling can be a useful tool for the a priori design of tDCS electrode positions, for a given study ( ).
Once desired locations are identified, the electrode assembly must be affixed to the head for delivery of current. Nonconductive headgear, used to position the electrodes on the body or scalp (e.g., elastic straps), are not included in the electrode assembly, but are critical for appropriate electrode placement ( ). For tDCS, using sponge-covered electrodes, elastic straps are traditionally used headgear for electrode placement. They require manual measurement of each electrode position, at each session ( Fig. 135.5,A2 ) and are typically adjusted on the head in an ad hoc manner ( Fig. 135.5,A3 ). If these straps are not properly set-up, electrodes may move over the course of a tDCS session. Thus, the distribution of current delivery changes over the duration of a tDCS session ( ), which undermines tDCS tolerability and replicability ( ). Moreover, if electrode straps are overtightened, there is an increase in the probability of evacuation of saline from the electrode sponges. For this reason, poorly designed head hear (such as neoprene caps) are not recommended for sponge-based tDCS, where fluid may run under the cap, leading to current flow distortion. Updated tDCS approaches can use headgear where the locations of the electrodes are preset (in this case each montage will have an associated headgear ( Fig. 135.5,B2 )). This type of headgear then simply slides onto the subject’s head, which also simplifies setup and increases consistency ( Fig. 135.5,B3 ).
Computational Models of Transcranial Direct Current Stimulation for Electrode Placement
tDCS is a single, programmable, electrotherapy device that can be simply configured to provide a diversity of dosages. Though this flexibility underpins the utility of neuromodulation, the myriad of potential dosages (e.g., stimulator settings and combinations of electrode placements) can lead to a great number of possibilities, thus making the optimal choice very difficult to readily ascertain. The essential issue in dose design is to relate each externally, controlled dose with the associated, brain regions targeted (and spared) by the resulting current flow and hence, the desired clinical outcome. Computational forward models aim to represent a critical tool in the rational design, interpretation, and optimization of neuromodulation ( ).
Common Montages
Primary motor cortex (M1) (Anode)-Supraorbital (SO) (Cathode): this is the most common tDCS montage. M1 is a target that modulates the sensory and motor, subthalamic activity, associated with chronic pain. It has been shown that the cortical excitability can be changed up to 40% ( ). The polarity (anode/cathode) of the electrode refers to the M1 electrode. Anodal stimulation results in neuronal, soma depolarization and increasing neuronal excitability, while cathodal stimulation has opposite results ( ). Only one motor cortex is stimulated, so that bilateral montages are an alternative for bilateral pain syndromes ( ). Potential confounding effects of the supraorbital electrode needs to be considered.
Dorsolateral Prefrontal Cortex (DLPFC) (Anode)-SO (Cathode): Is commonly tested for the treatment of depression ( ) and chronic pain ( ). This montage has yielded encouraging results ( ). Typical montages are bilateral DLPFC stimulation with the anode over the left DLPFC, but unilateral montages are possible ( ).
4 × 1 High-Definition (HD)-tDCS: Experimental results demonstrate the neurophysiological efficacy of HD-tDCS on motor cortex plasticity. 4 × 1 HD-tDCS, as well as other HD deployments, may offer the opportunity to induce plasticity more focally than conventional tDCS protocols ( ). Most published studies on 4 × 1 HD-tDCS have targeted the primary motor cortex (M1), particularly for pain-related outcomes ( ). However, there is a study that DLPFC was targeted also ( ).
Methods for the Generation and Use of Computational Forward Models of Transcranial Direct Current Stimulation
Computational models of tDCS range in complexity from concentric, sphere models to high-resolution models, based on an individual’s MRI. The appropriate level of modeling detail depends on the clinical question, being asked (as well as the available computational resources). Whereas simple geometries (e.g., spheres) may be solved analytically, realistic geometries employ specialized software, as described later, that include numerical solvers (namely finite element methods [FEM]). Regardless of complexity, all forward models share the primary outcome of correctly predicting brain-current flow during transcranial stimulation to guide clinical, therapeutic delivery.
For clinicians interested in using computational forward models to inform study design or interpretation, several options are available: (1) a collaboration with a modeling group or a company can allow for customized exploration of montage options (2) referencing existing published reports or databases for comparable montages (3) by utilizing a recently developed process, where a desired brain target can be selected and the optimized stimulation electrode montage is proposed within seconds ( ). If tDCS continues to emerge as an effective tool in clinical treatment and cognitive neuroscience, and concurrent modeling studies emphasize the need for rational (and in cases of patient-specific) dose decisions, then it will become incumbent for clinical research teams to understand the applications (and limitations) of computational forward models ( ).
While the specific software applications used can vary across modeling groups, in general, the approach and work-flow for model generation follows a similar pattern. The steps for generating high-resolution (anatomically specific) forward models of noninvasive neuromodulation are adapted from extensive, prior work on computational modeling. These involve various steps:
Step 1, demarcation of individual, tissue types (masks) from high-resolution, anatomical data, using a combination of automated and manual segmentation tools. It is worth noting that the respective contribution of the automated/manual interventions depends on (a) sophistication of the particular automated algorithm employed, since they are usually not optimized for forward, transcranial modeling ( ) and (b) the need for identification of anomalies, in suspect populations like skull defects, lesions, shunts, and so on. Consequently, as emphasized later in this section, the number and precision of the individual masks obtained is pivotal for the generation of accurate, three-dimensional (3D) models to capture critical, anatomical details that may influence current flow.
Step 2, modeling of the exact, physical properties of the electrodes (e.g., shape and size) and precise placement within the segmented image data (i.e., along the skin mask outer surface).
Step 3, generation of accurate meshes (with high-quality factor) from the tissue/electrode masks, while preserving resolution of subject and anatomical data.
Step 4, resulting volumetric meshes are then imported into a commercial, finite element solver.
Step 5, at this step, resistivity is assigned to each mask and the boundary conditions are imposed. The standard Laplacian equation is solved using appropriate numerical solver and tolerance settings ( ).
Step 6, data are plotted as induced, cortical electric fields or current density maps.
As head size and shape vary from person to person, it is important to use a method for common localization of electrode position. There are several methods for addressing this issue:
- 1.
international 10–20 (or 10–5) electrode placement system ( ), or another, gross anatomical, coordinate system ( ),
- 2.
neuronavigation systems (e.g., MRI guided; ), or
- 3.
physiology-based placement (e.g., TMS generated MEPs). At present, physiology-based placement can only be performed for motor and other primary cortices (e.g., sensory). However, further options may become available in the future (e.g., Transcranial magnetic stimulation (TMS)-electroencephalogram (EEG) methods). Regardless, these methods can be used to reproducibly center each electrode on the head, accommodating varied head shape or size ( ).
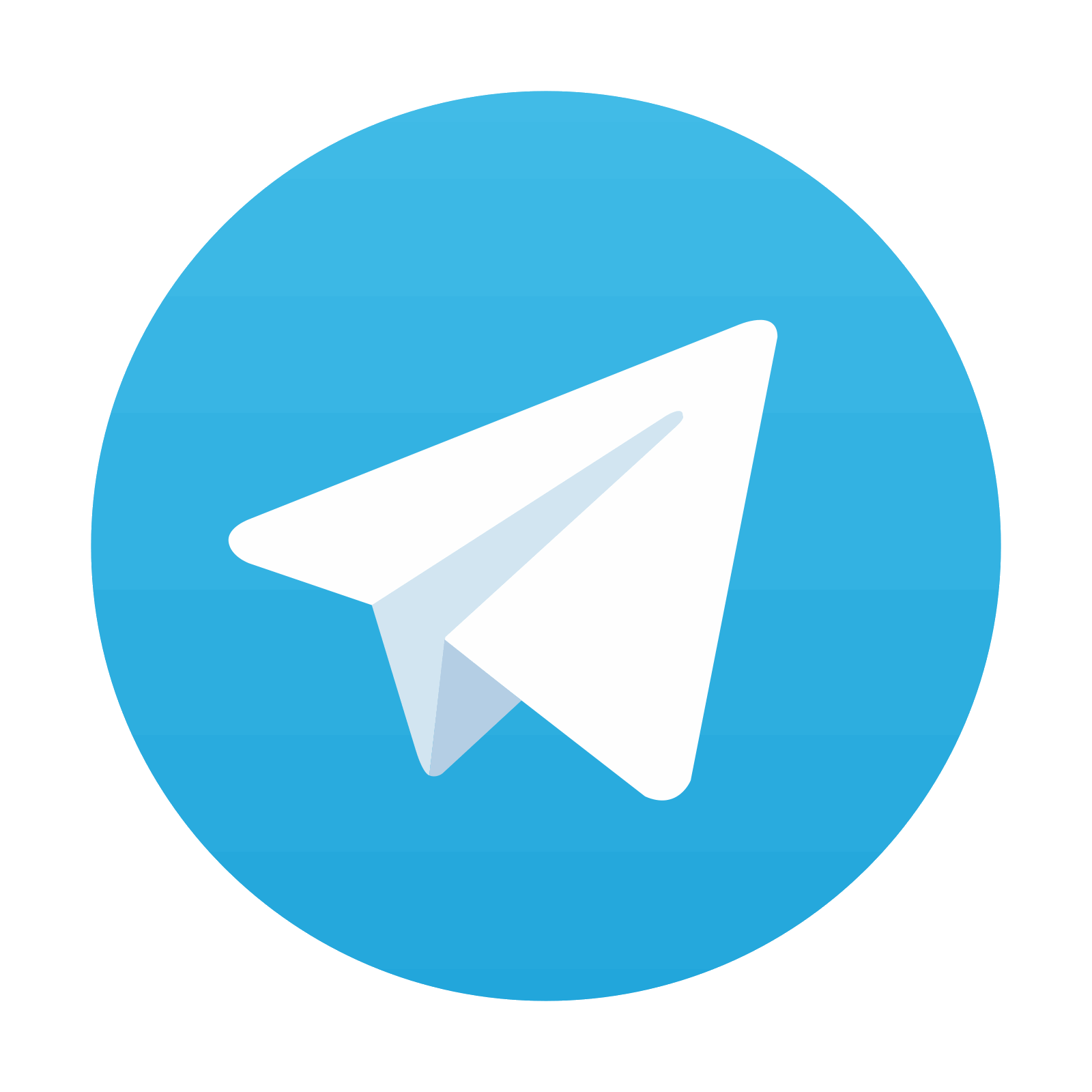
Stay updated, free articles. Join our Telegram channel
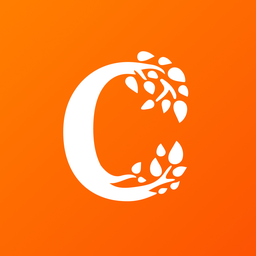
Full access? Get Clinical Tree
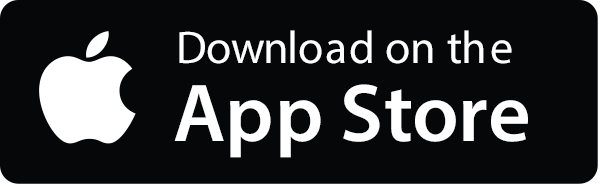
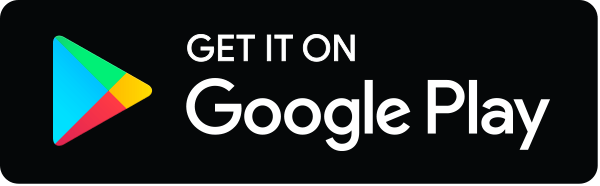