Abstract
More than 30 years have already passed since the seminal article of Baker et al., in which a transcranial magnetic stimulation (TMS) pulse over the motor cortex (MC) was successfully used to painlessly elicit a recordable electromyography response. Since then, multiple studies have been conducted exploring the underlying physiology of TMS effects, its potential uses in clinical settings, as well as safety. In this chapter, we provide an overview regarding the basic mechanisms of TMS, how it can be used as a tool to investigate human brain cortex physiology, how it can be used to alter cortical excitability, its safety concerns, and its use in clinical settings.
Keywords
Change cortical excitability, Clinical usefulness, Probe cortical excitability, TMS, Transcranial magnetic stimulation
Outline
Brief Introduction 1577
Basic Mechanisms of TMS and TMS as a Tool to Investigate Human Brain Cortex Physiology 1577
Distance Between the Coil and the Cortex 1578
Coil Orientation and Shape 1578
Transcranial Magnetic Stimulation to Probe Cortical Excitability, Inhibitory, and Facilitatory Mechanisms 1578
Single Pulse Transcranial Magnetic Stimulation 1579
Transcranial Magnetic Stimulation to Change Brain Activity 1580
Traditional Repetitive Transcranial Magnetic Stimulation 1580
Clinical Applications of Repetitive Transcranial Magnetic Stimulation 1581
Safety of Transcranial Magnetic Stimulation 1584
Conclusions 1585
Acknowledgments [CR]
References 1585
Acknowledgments
Authors are grateful to Melanie French and Jesse Gaboury for their help reviewing the final version of this chapter.
Brief Introduction
In , Anthony Barker et al. published in Lancet the first report of a new contactless and painless method for stimulating the motor cortex (MC). This novel method used a device to deliver a magnetic pulse over the MC to elicit a recordable electromyographic (EMG) response over the contralateral abductor digit minimi . This was not the first time that cortical stimulation, using noninvasive methods was performed. Indeed, in Merton et al. had already successfully used skin electrodes to induce electrical currents in the brain and the spinal cord, but this was the first time that a magnetic stimulator was used to induce a pain-free, muscle-evoked potential (MEP) ( ). This was the first report of the use of transcranial magnetic stimulation (TMS). In the following 30 years, TMS has evolved to a more refined technique, with multiple diagnostic and therapeutical uses. In this chapter, we will review the basic mechanisms of TMS and its safety profile, and provide a brief overview regarding its clinical diagnostic and therapeutic utilities as well as how it can be used to understand brain and behavior.
Basic Mechanisms of TMS and TMS as a Tool to Investigate Human Brain Cortex Physiology
The effect of TMS can be understood, based on the electromagnetic induction principle, proposed in 1831 by Michael Faraday. Electrical current flowing over a stimulation coil creates a changing magnetic field that is able to induce current flow over nearby conductors. In TMS, the current that flows through the coil generates an electromagnetic field that is able to induce eddy currents in a nearby neuron population. Please note in Fig. 134.1 that eddy currents (A) have the opposite direction to the ones (B) that generate them.

A TMS stimulator, in basic terms, is a high-current pulse generator that is able to produce a current discharge of thousands of amperes, which in turn is able to generate a brief magnetic pulse (around 100 μs) with a magnitude that can go up to several Tesla. If the coil is placed over the head, there is little attenuation to the magnitude of the pulse when it crosses tissues such as the scalp, cranial bones, meninges and liquor and therefore the induced electrical current is able to depolarize the underlying neuron population and induce effects over neural networks.
These TMS induced effects are modulated by several biological and physical parameters such as the distance between the coil and the target area, coil orientation and shape, pulse waveform, and several pulse parameters, such as intensity, frequency, latency between pulses, and pattern of stimulation, among others.
Distance Between the Coil and the Cortex
The cortex is an inhomogeneous conductor with conductivities ranging from 0.14 S/m (Siemens per meter) for the white matter to 1.79 S/m for the cerebrospinal fluid, while cranial bones have an estimated conductivity of 0.01 S/m ( ). So, the actual current density, induced by a TMS pulse, will be dependent on the tissue conductivity, but also on the distance between the coil and the stimulation target. Although the distance of a magnetic field is theoretically infinite, the common assumption is that it decays with the square of the distance. This limits the depth of TMS induced electrical fields to a few centimeters, usually between 0.9 and 3.5 cm ( ) and thus potentially more suitable for stimulating cortical, rather than subcortical regions. This fact is why some “deep brain” TMS coils were developed with the ability to deliver 81% of the maximal, induced, electrical field at skull, at a depth of 4.5 cm, when compared to 12% from the standard 70 mm figure-8 coil ( ).
Coil Orientation and Shape
The intensity of the magnetic field and the shape of the coil not only determine the depth of the induced electrical field but also the area of stimulation. Circular coils were the first ones to be used and are usually between 80 and 150 mm in diameter. Circular coils are not very focal, as the maximal, electrical current field is induced in the outer regions of the coil, with a maximum magnetic field in the center of the coil. Thus, the site of stimulation is not actually the center of the coil, but instead the regions surrounding the outer edge of the coil. Due mainly to its size, circular coils have a good penetration into the skull, and by placing them over the vertex, they can activate both hemispheres simultaneously. In the event of a monophasic pulse, the effects of such coils in the hemispheres will be asymmetric, mainly because the effect will be greater in the hemisphere in which the current flows in a posteroanterior direction ( ). The main caveat of such coils is its lack of focality, as the induced electrical field across the outer edge of the coil is pretty much similar all around.
To increase focality, figure-8 coils were developed. By placing two round coils side by side, the current will flow in the same direction at the junction point, which results in a summation of the electrical field and allows focal stimulation to a specific target. But the figure-8 coils are smaller (usually 70 mm) than circular ones, and thus, the resulting effect is a more modest penetration. Nonetheless, this claim has been challenged by the use of double cone, figure-8 coils, which have higher penetration in the skull than the figure-8 flat coils. Overall, figure-8 flat coils have a great compromise between focality and penetration and are the most common coils used for clinical purposes and research.
Transcranial Magnetic Stimulation to Probe Cortical Excitability, Inhibitory, and Facilitatory Mechanisms
TMS has been used to probe cortical excitability, facilitatory, and inhibitory cortical mechanisms, to indirectly infer about the underlying neurophysiologic neurotransmission processes and to alter cortical excitability and/or brain function. The effect of TMS will be dependent on several parameters such as pulse waveform, intensity of the electrical field, the number of pulses, as well as the interval between pulses and trains.
Single Pulse Transcranial Magnetic Stimulation
If a single pulse of TMS is delivered over MC, it can depolarize the motor neurons and cortical excitability can be probed noninvasively. If electrical current is delivered to peripheral nerves, it is possible to elicit an evoked electromyographic activity in the innervated group of muscles, or an MEP. Similarly, a single TMS pulse over the cortical representation of those muscles can be used to induce an evoked electromyographic response over that group of muscles. The resulting MEP can be recorded and cortical excitability can be inferred. The actual size of the MEP (i.e., amplitude) will not be a direct product of the stimulus intensity, but a combination of three factors: the number of recruited neurons, the number of neurons that will actually discharge more than once, and the TMS-induced discharge synchronization across motor neurons. This leads to an assumption that MEP amplitude then increases with TMS output intensity, which has been thought to represent increased corticospinal and spinal neural recruitment. Nonetheless, there is a considerable interindividual variation in the stimulus response variation. For instance, standardizing TMS to the resting motor threshold (RMT) (i.e., minimum intensity to elicit MEPs with 50 μV, peak-to-peak, in 50% of the trials) ( ) does not ensure that all subjects will reach the same MEP amplitude. And if the intensity is increased by 20%, the interindividual variability increases, ranging from 6% to 100% of MEP, at maximum amplitude ( ).
Cortical Silent Period
The cortical silent period is an interruption of voluntary muscle contraction following a TMS pulse over the contralateral MC. The first 50 ms of this interruption can be attributed to spinal mechanisms, but the latter portion (100 ms) seems to be of cortical origin ( ) and varies, depending on the intensity used, on the level of muscle contraction and also due to task difficulty ( ). This intracortical inhibition is thought to reflect γ-aminobutyric acid B (GABA B ) interneuron activity that synapse with pyramidal neurons ( ).
Paired Pulse
If two pulses, a conditioning stimulus and a test stimulus, are delivered in a quick succession, it is possible to study the effects of the conditioning stimulus on the brain. This technique is known as paired pulse and measures the effect of the conditioning stimulus (first pulse) on the response of the test stimulus (second pulse). Paired-pulse TMS can provide valuable insights about cortical excitability as well as about cortical inhibition and facilitation. Depending on the intensity of the conditioning stimulus and the interstimulus interval, the response on the brain can be either facilitation or inhibition.
Short-interval intracortical inhibition (SICI) was described first by . Two stimuli are applied to the hand area of the MC through the same coil in a quick succession. If the interval between pulses is very short (i.e., 1–5 ms) ( Fig. 134.2A ), the subthreshold conditioning stimulus will reduce the size of the subsequent, suprathreshold, test stimulus (normally adjusted to elicit a 1 mV peak-to-peak MEP). This suggests that this MEP reduction following TMS reflects a cortical inhibitory mechanism, as it does not reduce the amplitude of H-reflex 1
1 Reflex muscle reaction following electrical activation of sensory fibers.
, nor the amplitude of MEPs induced by electrical stimulation, which are thought to activate corticospinal neurons directly ( ). This strongly suggests that SICI is generated by synaptic inhibitory mechanisms at the level of primary MC interneurons.
Moreover, in the 1–5 ms range, I3 but not I1 waves 2
2 Fast rhythmic responses in motor pathways.
are suppressed, suggesting a GABAergic inhibition effect over the MC ( ). Also, allosteric modulators, at the GABA A receptor, have been shown to increase SICI (e.g., ), thus making single pulse TMS a suitable technique to probe MC excitability.Intracortical Facilitation
Fig. 134.2B represents an intracortical facilitation protocol. If the interval between the subthreshold conditioning stimulus and the test stimulus is increased to 10–15 ms, there is a facilitation of the test MEP. This facilitation is not associated with the H-reflex ( ) and has been suggested that it is mediated by glutamatergic, N -methyl- d -aspartate receptors (NMDAR). Although the physiology underlying intracortical facilitation is less understood than the one surrounding SICI, it is thought to be the product of weaker inhibition, with prevailing facilitation.
Short Intracortical Facilitation
If two pulses at motor threshold intensity or suprathreshold conditioning stimulus followed by a subthreshold stimulus (e.g., ) are delivered to the same motor region with discrete interstimulus intervals of 1.0–1.5 ms, 2.5–3.0 ms, and at ∼4.5 ms, there is an MEP facilitation. As this SICI shows the same periodicity as the corticospinal volley (interpeak intervals of ∼1.5 ms), it is thought to be dependent on I (indirect) wave generation 3
3 I-waves have a periodicity of approximately 1.2 ms ( ). Indirect waves are thought to originate from repetitive synaptic inputs to pyramidal neurons (layer 5) of motor cortex, with early I-waves being generated by synapses closer to the soma, and later ones by distal synapses ( ).
, following MC activation. Paired-pulse TMS at I-wave periodicity is able to induce an increase in the amplitude of the motor evoked potential by facilitating the interaction between the first pulse and second TMS pulses ( ).Long Interval Intracortical Inhibition
If two suprathreshold pulses are delivered 60–150 ms apart, there is an inhibition of the test MEP ( ). Although not totally understood, inhibition at later stages are thought to reflect cortical inhibition, especially because epidural recordings showed that I-waves (later waves) are inhibited at the 50–200 ms interval, while there is no change for the D waves (Direct waves) originated from axonal activation of corticospinal neurons ( ). Moreover, LICI is probably mediated by GABA B receptors, especially because bacoflen, a GABA B receptor agonist, has been shown to enhance LICI ( ). Therefore, paired-pulse protocols can be used to examine noninvasive, distinct inhibitory mechanisms, namely GABA A with SICI and GABA B with LICI. Moreover, LICI seems to reduce SICI, probably by a presynaptic GABA B receptor inhibition of the inhibitory interneurons involved in SICI ( ).
Paired Pulse to Probe Interhemispheric, Cerebellar, and Afferent Inhibition
If a conditioning stimulus is delivered ipsilaterally over the MC, and 6–15 ms later a test stimulus is delivered over the contralateral MC, there is a 50%–75% reduction in the test stimulus induced MEP ( ), likely mediated by transcallosal inhibition ( ). Similarly, a TMS pulse, applied 5–7 ms prior to the test pulse over the contralateral motor region, results in a MEP inhibition. A possible explanation for this phenomenon is that the Purkinje cell output results in a reduction of excitatory output from the deep cerebellar nuclei to the MC, which results in a MEP inhibition.
Changes in the cortex can also be achieved by changing peripheral nerve activity. For instance, pulses delivered to the median nerve before TMS over the contralateral MC are able to decrease the MEP amplitude at two stages. The first peak is around 20 ms. This short afferent inhibition (SAI) ( ) is thought to reflect cholinergic-dependent, GABA A receptor activity ( ), as Alzheimer’s Disease patients exhibit decreased SAI activity, which is restored by the use of cholinesterase inhibitors ( ). Moreover, in vivo studies in healthy volunteers have shown that scopolamine, a selective, muscarinic, cholinergic blocker, is able to reduce or abolish the SAI ( ), thus providing additional evidence for the SAI as a potential marker for probing cholinergic activity. The second peak is around 200 ms and this long afferent inhibition (LAI) is thought to be mediated by GABA B receptors ( ).
Transcranial Magnetic Stimulation to Change Brain Activity
Repetitive TMS (rTMS) applied to cortical regions can induce changes that outlast the stimulation period. This neuroplastic effect of rTMS has been used to understand the mechanisms underlying brain plasticity, to study brain function by momentary arrest of function, or as potential tool for clinical rehabilitation for several neuropsychiatric disorders. rTMS protocols can be divided into traditional, if the interstimulus interval is kept constant, and patterned if the interstimulus interval is variable.
Traditional Repetitive Transcranial Magnetic Stimulation
Traditional rTMS is US Food and Drug Administration (FDA) approved for mild treatment resistant depression and migraine. This type of rTMS can be divided into low frequency (LF) (<1 Hz) ( Fig. 134.3A ) or high frequency (HF) (>1 Hz) ( Fig. 134.3B ). Traditionally, LF rTMS has been thought to decrease cerebral blood flow (CBF) ( ), while HF has been thought to increase CBF ( ), which is related, respectively, to its effects of decreasing or increasing brain activity. Nonetheless, this monotonic relationship has been challenged, especially because different networks in the brain (e.g., visual and somatosensory) exhibit frequency-dependent curves ( ). Moreover, multiple sessions of rTMS have been shown to outlast the stimulation period with after effects that last up to 6 months, depending on the disease treated. The classic interpretation of how rTMS works is based on its effects on action potentials that can change synaptic plasticity to induce long-term potentiation (LTP) and/or long-term depression (LTD). These changes can modulate neural networks and corticocortical connections, which are correlated to functional improvement. In addition, rTMS may also modulate the spontaneous oscillatory rhythms of cortical circuits.

Other possible mechanisms may include changes in gene expression, enzyme activity, and neuromediator production (e.g., ). Furthermore, rTMS seems to have a neurotrophic effect and influence over concentration of brain-derived neurotrophic factor (BDNF) ( ). Another possible effect that is less explored relates to the biophysical effects of magnetic fields of the brain ( ).
Patterned Repetitive Transcranial Magnetic Stimulation
Patterned rTMS is a generic name to designate protocols with different interstimulus intervals. Theta burst stimulation (TBS) is considered a type of patterned rTMS because it consists of three pulses given at 50 Hz (20 ms interstimulus interval) in 5 Hz bursts (i.e., Theta). If applied continuously (continuous TBS, cTBS) during 20 (300 pulses) or 40 s (600 pulses) ( Fig. 134.4A ) at 80% active motor threshold activity is able to decrease cortical excitability. If applied intermittently (iTBS), during 2 s every 10 s for a total of 190 s (600 pulses) ( Fig. 134.4B ), TBS is able to increase cortical excitability. TBS is especially interesting because it mimics the well-known effects of LTP or depression LTD 4
4 Long-term potentiation (LTP) refers to the process of activity dependent plasticity that results in synaptic strengthening, while long-term depression (LTD) refers to the long lasting reduction of synaptic activity.
found in animal physiology studies ( ). Additionally, TBS is administered for a short duration of time, but has a very long, after effect period, when compared to other rTMS techniques (see for review).
Paired Associative Stimulation
TMS is thought to activate postsynaptic pyramidal outputs via horizontal intracortical fibers, while somatosensory information is thought to converge on pyramidal cells within the MC ( ). This may explain the PAS effects, as long-lasting changes in the MC can be achieved by a coactivation mechanism in which afferent, median nerve stimulation is combined with transcranial direct current stimulation to the MC—spike-timing-dependent plasticity. With 25 ms difference between median nerve stimulation and TMS over the cortex, there was a long lasting (over 60 min) increase of 50% in the MEPs recorded from the resting APB ( ). If the interval is changed from 25 (i.e., PAS 25 ) to 10 ms (i.e., PAS 10 ) instead of facilitation, PAS results in an MEP depression ( ). This suggests that timing of events is critical for the PAS effects: with 25 ms the events in the MC follow those induced by the median nerve stimulation, while with 10 ms, the events are reversed.
Clinical Applications of Repetitive Transcranial Magnetic Stimulation
In the last decade, TMS has also been used as a therapeutic technique. For therapy, as discussed earlier, repetitive pulses are used in order to induce changes in the nervous system, in a technique known as repetitive transcranial magnetic stimulation (rTMS). The basis for this application is mainly founded on the concept that LF (<1 Hz) pulses induce decreased cortical excitability, whereas HF (>5 Hz) pulses cause increased cortical excitability.
However, recent studies show that this concept may be oversimplified and the effect of rTMS may vary based on different aspects such as rTMS parameters (intensity, interval, and duration of the stimulus), individual characteristics of the patient (gender, age, and genetic aspects), and especially baseline neuronal dysfunction, which can influence homeostatic plasticity and metaplasticity.
Overall, there many different mechanisms of rTMS that are being explored at various levels. However, all point to its therapeutic potential to treat multiple diseases, such as depression, chronic pain, stroke, epilepsy, and Parkinson’s disease. The method of stimulation over the cortex varies depending on specific aspects of diseases.
Depression
Depression is the first condition to be treated by rTMS and remains the most studied clinical uses of rTMS. Pascual-Leone et al. and George et al. were the first to show that HF rTMS over the left dorsolateral prefrontal cortex (DLPFC) can improve depressive symptoms ( ). The basis for this approach arose from previous studies that used electroencephalographic (EEG) and functional brain imaging (fMRI) to show that depressed patients have asymmetrical frontal lobe activity, with a hypometabolic state in left frontal regions and concomitant hypermetabolic states in the right prefrontal regions.
Later, , showed that LF rTMS over the right DLPFC also has an antidepressant effect. In this approach, the LF is inhibitory, based on the hypothesis that depression is correlated with hyperactivity in the right DLPFC. Many other studies came after this, confirming the DLPFC region is an important cortical target for noninvasive brain stimulation. Among the reasons for the DLPFC to be such an important target is that the DLPFC is an accessible “hub” of cortical connections between frontal cortex and other structures involved with the limbic system and mood regulation; in this way, stimulating DLPFC can modulate the entire network.
Subsequently, several high-quality randomized control trials (RCTs) have supported HF left DLPFC stimulation as having an antidepressant effect, raising support to meet the criteria of level “A” evidence. In a meta-analysis that included 29 studies, totaling 1371 patients ( ), the average rate of responders was 29% of patients. Although HF rTMS over the left DLPFC is the most studied parameter to treat depression, LF rTMS has also yielded good results. In another meta-analysis performed by the same author, eight studies were identified totaling 263 patients who used LF rTMS over right DLPFC ( ), with an average rate of responders being 38%. A separate meta-analysis from compared the two approaches and showed a similar effect of HF left DLPFC when compared to LF right DLPFC.
Another possible approach is the combination of HF left DLPFC and LF right DLPFC. , were the first to test this method against unilateral intervention, showing no difference between bilateral stimulation (i.e., HF to the left and LF to the right DLPFC), when comparing to two unilateral conditions (i.e., to the left DLPFC): HF alone or alternating between LF and HF trains. In a meta-analysis of 7 RCTs, testing bilateral intervention, concluded that bilateral rTMS seems to be effective; however, it is not clear how the response compares to unilateral intervention.
In the United States, the FDA cleared the first TMS device in 2008. According to the US “Clinical TMS Society” (cTMSs), the standard rTMS protocol may vary and depends on product labeling, but approved parameters are HF (10–18 Hz) over left prefrontal cortex, applied during five daily sessions per week for 4–6 weeks total. That being said, an additional 1–4 weeks may benefit patients that are slow responders. The labeled indication of TMS therapy is: “ TMS therapy is indicated for the treatment of Major Depressive Disorder in adult patients who have failed to receive satisfactory improvement from prior antidepressant medication in the current episode. ”
In one publication, the US cTMSs confirmed recommendations for the routine use of TMS in clinical practice that follows the format of the Grades of Recommendation framework published by the University of Oxford Center for Evidence Based Medicine. Among these recommendation included as strength “ A ”: (1) “TMS therapy is recommended as an acute treatment for symptomatic relief of depression in the indicated patient population”; (2) “TMS therapy is recommended for use as a subsequent option in patients who previously benefited from an acute treatment course and are experiencing a recurrence of their illness (continuation or maintenance)”; (3) “TMS therapy can be used as a continuation or maintenance treatment for patients who benefit from an acute course.” The recommendation for strength “ B ” is : (4)“TMS therapy can be administered with or without the concomitant administration of antidepressant or other psychotropic medications”; (5) “TMS therapy can be reintroduced in patients who are relapsing into depression after initially responding to TMS treatment.”
Regarding safety concerns, although the overall risk of seizure is estimated to be less than 1%, it is important to have a method for prescreening eligible patients to assess potential seizure risk. Additionally, clinical personnel involved in TMS application must be trained as first responders in order to provide proper initial management of a seizure or other medical event.
Although TMS treatment for depression is already being used in several countries, an obstacle to its broad dissemination is that is requires daily sessions that take place over the course of several weeks. In most cases, this requires the patient to be transported to the treatment site, which potentially results in a higher cost than pharmacotherapy.
Pain
Pain is commonly defined as an “unpleasant sensory and emotional experience associated with actual or potential tissue damage, or described in terms of such damage” ( ). This definition is in agreement with the pain model proposed by Melzack in , called “Neuromatrix,” in which pain is divided into three spheres: sensorial-discriminative, affective-motivational, and cognitive-evaluative. Currently, a growing body of evidence supports that pain is the result of supraspinal cortical processing and its experience results from the way the brain processes sensory stimuli, rather than the sensory stimuli itself.
Acute pain is a physiologic sensation and is a warning of actual or potential tissue injury, which usually disappears after a disruption of the painful stimulus. However, pain may become chronic (either continuously or episodically), especially if acute pain is not properly treated. Therefore, chronic pain may be seen as maladaptative changes in nervous system.
Chronic pain is highly prevalent in the general population, with an estimated 12-month prevalence of 37% in developed countries ( ). Its treatment is often a major challenge in clinical practice, with common failure of therapeutic response and intolerance to medication use. In , Tsubokawa et al. noninvasive brain stimulation was shown to have analgesic effects in chronic neuropathic pain conditions by placing electrodes over the MC. This type of stimulation is referred to as epidural MC stimulation. A decade later, showed similar pain relief improvement in chronic neurogenic pain using HF rTMS (but not LF rTMS) over the MC. Further studies have shown a reduction in pain using rTMS over the MC in various conditions including brachial plexus injury, complex regional pain syndrome, type I, peripheral nerve injury, and spinal cord injury. Given these results, rTMS provides a safer alternative to surgical treatment. It also has been shown to predict the response of MCS, since patients who improve with rTMS are more likely to improve with MCS.
In addition to the MC, DLPFC stimulation seems to be beneficial for neuropathic pain, fibromyalgia, and postoperative pain improvement in gastric surgery using similar parameters that are used to treat depression (i.e., HF in the left DLPFC or LF in the right DLPFC) (e.g., ). The motivation for choosing the DLPFC is due, in part, to great relationship between chronic pain and depression, as disabling chronic pain is present in 41% of patients with depression (comparing to only 10% in patients without major depression disorder) ( ).
Another possible target for rTMS to treat pain is the right somatosensory secondary cortex (SII), as a study showed an improvement of visceral pain caused by chronic pancreatitis with the frequency of 1 Hz ( ). The effect of rTMS appears to be specific to the stimulated cortical region, since its use in the supplemental motor area, premotor area, and primary somatosensory cortex did not show a benefit for pain management. Though this was a small study ( ) and other studies need to confirm these findings.
In one review, HF rTMS applied to the MC, contralateral to the pain side for neuropathic pain conditions had level A (definite) evidence. On the other hand, LF rTMS applied to the contralateral MC on the side of the pain had level B (probable) evidence for being ineffective for treating neuropathic pain ( ). Therefore, it is not only important to identify the location of the stimulation, but also other parameters, such as frequency.
Among the studies using HF rTMS, other parameters varied such as number of sessions (ranged from 1 to 10), the number of pulses per session (ranged between 500 and 2000) and the intensity (varied between 80% and 95% of the motor threshold) (e.g., ). It is not known what are the best stimulation parameters. A single session has shown to produce analgesic effects that can last for a few days, and it is believed that the effect may be longer with a larger number of sessions, showing rTMS can be a potential instrument used in clinical practice. For nonneuropathic pain, such as fibromyalgia, visceral pain, and migraine, there are studies showing improvement of pain relief (e.g., ), however with a smaller level of evidence.
Although several mechanisms are involved with chronic pain (such as ectopic excitability, structural reorganization, primary sensory degeneration, disinhibition and peripheral sensitization, and central sensitization), evidence has suggested that both excitatory and inhibitory synaptic plasticity is related to the mechanism of chronic pain (see for review). Another possible mechanism to explain the modification of acute to chronic pain presupposes that pain has the potential to induce long-term memories ( ), reinforcing learning mechanisms. In this way, chronic pain is seen as a result of maladaptive plasticity or a pathological learning, and rTMS is a way of modifying such connections, producing analgesia.
The application of rTMS to the primary MC (M1) induces an electrical current that directly activates local neurons that indirectly act in distal structures (such as the dorsal pre-MC, supplemental motor area, primary somatosensory cortex, and cingulate motor area), as well as deeper regions (such as basal ganglia and cerebellum). Thus, stimulation of the MC can act on GABAergic thalamic circuits through the corticothalamic pathways, inhibiting the hyperactivity of thalamic nuclei, thus blocking the nociceptive and somatosensory stimuli, and likely activating the opioid system via the periaqueductal gray matter ( ).
In this manner, M1 might be more related to the sphere of sensorial discrimination of pain, although there is the possibility of the connection of M1 with the limbic system, also influencing the affective-emotional component of pain. It is proposed that rTMS over the DLPFC modifies mostly the pain but not sensory threshold. It is possible that rTMS over DLPFC acts through the connections in the corticostriatal-thalamic-cortical loop ( ), reducing the perception of nociceptive stimuli, and modulating the affective and cognitive component of chronic pain. This can attenuate the sensation of discomfort.
Additionally, the secondary somatosensory cortex (IBS) may also be modulated by rTMS ( ). The IBS is an important region of the brain involved in sensorimotor integration, as well as attention related to pain, learning and memory, including visceral and possibly nonvisceral nociceptive processing.
Stroke
In 2005, studies from , and , showed an improvement of motor function by LF rTMS over the contralesional MC and , by applied HF rTMS of the ipsilesional MC.
The rationality for these methods of stimulations is based on the interhemispheric imbalance theory, which theorizes that the inhibition that the lesioned hemisphere exerts on the unlesioned hemisphere decreases due to loss of neurons and decrease of excitability in perilesional areas. The increase of the activity in the contralesional hemisphere will inhibit, even more, the lesioned hemisphere via transcallosal projections to homologous regions. This imbalance of cortical excitability is likely a result of maladaptive plastic changes that prevent perilesional areas from recovering the function of the lesioned hemisphere. Therefore, the increasing cortical excitability in the lesioned hemisphere with HF rTMS or decrease cortical excitability with LF rTMS may help the brain to restore the interhemispheric imbalance and allows the “unmasking” cerebral areas that can help to recovery the impaired function. Although there are studies suggesting that, in some patients at least, the increased activity in the contralesional hemisphere may help to restore function, to date, most rTMS studies for stroke motor function improvement are based on this interhemispheric imbalance theory ( ).
The most studied situation in stroke is rTMS applied over the MC to improve motor function (as described earlier). Although there are almost 20 placebo-controlled trials including more than 500 patients, the use of rTMS for motor function recovery in stroke patients does not reach the level of evidence “A” ( ). One of the reasons for this is that most of the trials are exploratory, testing different parameters of stimulation and the association with others rehabilitation therapies. Moreover, it is likely that the effect of rTMS depends on patient’s characteristics, such as time since the stroke (acute, subacute, or chronic phase), type of lesion, age, among others. Still, the use of LF rTMS in the contralesional MC of chronic stroke patients can be considered level “B” of evidence ( ).
Besides motor function, there are studies showing that rTMS can be beneficial for others deficits after stroke, including aphasia (Broca’s and Wernicke’s), dysphasia, hemispatial neglect (e.g., ). In these situations, the methods most explored are based also on the notion of interhemispheric imbalance.
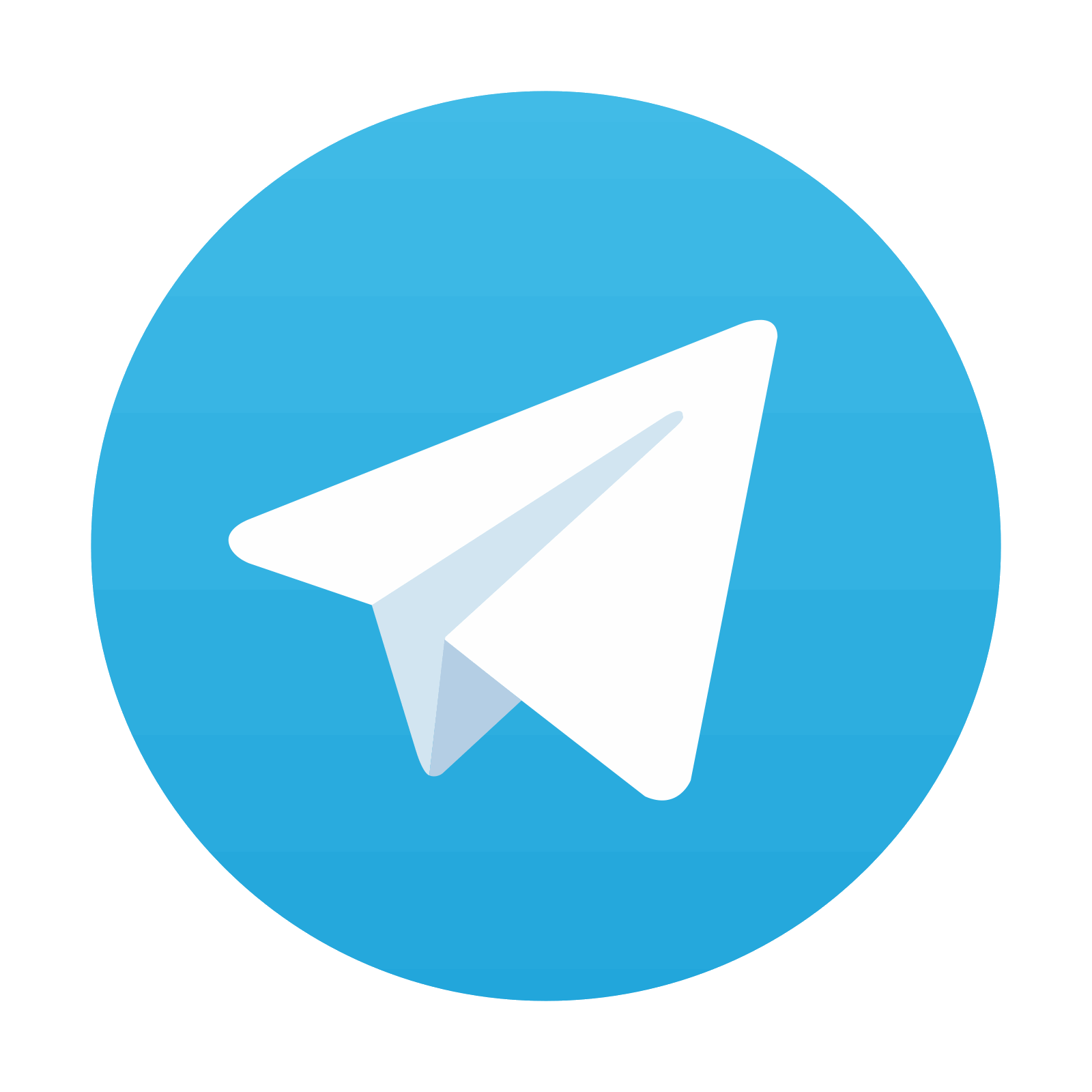
Stay updated, free articles. Join our Telegram channel
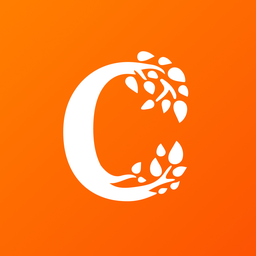
Full access? Get Clinical Tree
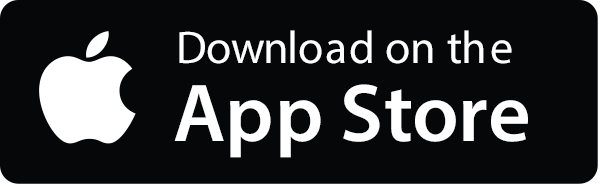
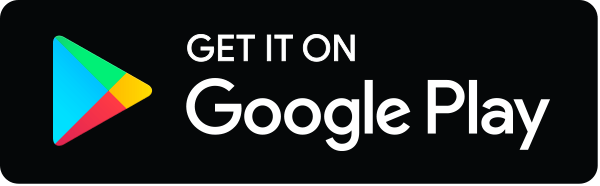