Abstract
There has been a steady increase in the amount of published data on the mechanism of action (MOA) of vagus nerve stimulation (VNS) in the past 2 decades, but the exact therapeutic mechanisms remain to be elucidated. Nevertheless, significant progress has been made, and the authors will discuss this evidence in both a fundamental way and from a translational perspective. Following an overview of the anatomy and the history of VNS, the discussion of the MOA of VNS is sequentially divided into electrical stimulation of the vagus nerve and how stimulation parameters affect the activation of vagus nerve fibers. The following sections discuss various central effects of VNS in relation to activation of cervical vagal afferents, and the final sections concern effects predominantly ascribed to the activation of vagal efferents. The most important MOA pathways are schematically represented.
Keywords
Depression, Epilepsy, Fibers, Mechanism of action, Vagus nerve, Vagus nerve stimulation
Outline
Anatomy of the Vagus Nerve 211
The History of Vagus Nerve Stimulation 212
Electrical Activation of Vagus Nerve Fibers 212
The Nucleus of the Solitary Tract as a Relay for Central Effects of Vagus Nerve Stimulation 213
Anticonvulsant Effects of Vagus Nerve Stimulation 214
Antidepressant Mechanisms 216
Analgesic Mechanisms 216
Mechanisms Underlying Effects of Vagus Nerve Stimulation on Cognition 216
Cardiac Effects of Vagus Nerve Stimulation 217
Anti-Inflammatory Effects of Vagus Nerve Stimulation 217
Conclusions 218
References 218
Anatomy of the Vagus Nerve
The vagus nerve is the 10th cranial nerve, which is a mixed nerve consisting of approximately 80% afferent and 20% efferent fibers ( ). A schematic overview of the anatomic pathways is provided in Fig. 18.2 . The nerve exits the brainstem bilaterally at the level of the medulla oblongata and leaves the skull through the foramen magnum to run in parallel with the carotid arteries ( ). The cervical part of the vagus nerve gives off several branches, which provide sensory and parasympathetic innervation to the heart, lungs, and esophagus ( ). Two other vagus nerve branches are relevant to the clinical application of vagus nerve stimulation (VNS). The recurrent laryngeal nerve branches off caudally to the aortic arch to innervate the laryngeal muscles. Coactivation of this branch during VNS may cause typical side effects such as hoarseness and a tingling throat sensation in patients ( ). It also provides the anatomical basis of laryngeal muscle evoked potentials (LMEPs), which have been recorded with VNS ( ) and can potentially serve as noninvasive biomarkers for fiber activation. The auricular branch of the vagus nerve (ABVN) carries sensory afferents of the auricular concha and most of the area around the auditory meatus; the ABVN travels alongside vagus nerve fibers. This ABVN is used in transcutaneous auricular VNS (tVNS) ( ), a recently developed, noninvasive, neurostimulation technique. The vagus nerve passes through the diaphragm alongside the esophagus and gives off several subdiaphragmatic vagal branches, which provide sensory and parasympathetic innervation to the remaining parts of the gastrointestinal (GI) tract, the pancreas, the liver, and the adrenal glands ( ).
The cell bodies of primary vagal afferents reside in the nodose and jugular ganglia located just outside of the skull and project predominantly to the nucleus of the solitary tract (NTS) bilaterally in the brainstem ( ). These afferents transmit sensory information of several modalities, including blood pH and blood pressure, that are sensed by chemoreceptors and baroreceptors in major arteries, as well as sensations like hunger, satiety, and nausea, based on sensory information arising from the GI tract ( ). An estimated 80%–90% of vagal afferents are unmyelinated, multimodal C-fibers, conveying mainly chemical information ( ). The remaining 10%–20% are B- and A-fibers that convey mainly mechanical information ( ). The vast majority of efferent, parasympathetic fibers are thinly myelinated or unmyelinated B- or C-fibers that arise from the dorsal vagal motor nucleus and the nucleus ambiguous, with the exception of the thick, myelinated motor fibers, innervating the laryngeal muscles ( ).
The History of Vagus Nerve Stimulation
The oldest record of VNS in the PubMed database dates back to 1886 ( ), describing mechanical stimulation of the vagus nerve via carotid massage to halt seizures. Though early animal experiments showed desynchronization of brain rhythms along with attenuation or elimination of strychnine-induced spike activity during electrical VNS ( ), it was only in the late 1980s that VNS was systemically investigated in experimental seizure models ( ). Convincing outcomes of these studies led to the first clinical implantation of a VNS device in 1988 ( ) and full clinical development and regulatory clearance of VNS for the treatment of drug-resistant epilepsy by the European Medicines Agency in 1994 and by the US Food and Drug Administration (FDA) in 1997. The finding of mood improvement in patients with epilepsy treated with VNS ( ) led to clinical trials for depression and the approval of VNS for drug-resistant depression in 2005. Today, more than 100,000 patients are being treated with VNS.
Electrical Activation of Vagus Nerve Fibers
Clinical VNS is applied to the fibers in the cervical trunk of the left vagus nerve ( Fig. 18.1A ). Electrical activation of axons will, under most circumstances, result in a bidirectional conduction of action potentials (APs), although in most cases only the orthodromically conducted potentials are effective. The pair of helical electrodes that are surgically implanted around the cervical vagus nerve for clinical applications in VNS has the anode placed caudally to induce an anodal block of efferent APs and thus prevent side effects due to activation of parasympathetic vagal efferent fibers. An anodal block refers to the fact that an electrical stimulus of positive polarity will hyperpolarize the axons and attenuate or even block the generation of APs for a short period of time. Preclinical evidence, however, suggests that this concept is mostly theoretical ( ). When electrically stimulating nerves, nerve fibers are recruited in an orderly fashion, with large-diameter, thickly myelinated A-fibers being recruited at the lowest intensities, thinly myelinated B-fibers being recruited at intermediate intensities and thin, unmyelinated fibers being recruited at the highest intensities ( ). The pulse intensity is a function of the pulse width and the output current. A study in dogs ( ), where the size and geometry of the vagus nerve are comparable to those of humans, found that afferent A-fibers were recruited at a mean threshold of 0.37 ± 0.18 mA, fast B-fibers at a threshold of 1.6 ± 0.36 mA, slow B-fibers at a threshold of 3.8 ± 0.84 mA, and C-fibers at a threshold of 17 ± 7.6 mA, using a pulse width of 300 μs. Additionally, thick, myelinated, laryngeal motor fibers were recruited at a threshold of 0.36 ± 18 mA, similar to the threshold for recruitment of type A afferents. Interestingly, LMEPs in rats were found to be recruited at a threshold of around 0.3 mA ( ), suggesting that these intensities, despite differences in stimulation geometry, may be comparable across smaller and larger species.

Though initial evidence suggested the necessity of recruiting C-fibers, in order to produce anticonvulsant effects of VNS, due to the high stimulation intensities used (5–15 mA, 500-μs pulse width) ( ), more recent studies have found anticonvulsant effects using much lower intensities (<1 mA, 250–500 μs pulse width) ( ). The observation that neonatal capsaicin treatment, which eliminates C-fiber afferents, had no influence on the anticonvulsant effects of VNS ( ) further supported the predominant involvement of type A- and B-fibers. In rats, VNS has been found to affect brain physiology and excitability at intensities as low as 250 μA ( ), which theoretically rules out a role for B-fibers.
Recent lines of evidence, discussed later, have focused on using VNS to regulate both the heart and the immune system by stimulating B-fiber efferents. This requires higher stimulation intensities than those used to modulate brain physiology, which indeed is suggested by a previously published study ( ).
Important parameters for the effectiveness of activation of vagal fibers include both output current amplitude and pulse width. Commercially available VNS systems, however, also allow adjustment of both pulse frequency and duty cycle. Using a higher pulse frequency may result in a higher rate of generation of APs. When the frequency is sufficiently high, this may summate postsynaptically and potentially augment associated effects. Early preclinical studies showed that pulse frequencies of 20–30 Hz were superior to lower frequencies ( ), while high pulse frequencies (>50 Hz) have been associated with irreversible nerve damage and therefore are not recommended for clinical applications ( ). For this reason, most clinicians use a pulse frequency in the 20–30 Hz range. Studies on duty cycle efficiency remain relatively sparse. The use of duty cycles for VNS is based on the observation that anticonvulsant effects in preclinical experiments were found to outlast the active stimulation period. Although there is no clear evidence to support its use, the most commonly used duty cycle in the clinic is 30 s ON/300 or 600 s OFF. Some retrospective studies have argued that duty cycles with a higher percentage ON-time provide little additional effect ( ), but it must be noted that these studies generally have been confounded by the tendency to treat the most resistant patients with more intense VNS duty cycles. Recent preclinical observations on the other hand suggest that a more intense rapid VNS duty cycle (7 s ON/18 s OFF) had a greater impact on brain physiology than a lighter, more clinically used standard VNS duty cycle (30 s ON/300 s OFF) ( ). The current guidelines for selection of VNS parameters are thus based mainly on empirical clinical evidence, warranting larger systemically conducted trials.
The Nucleus of the Solitary Tract as a Relay for Central Effects of Vagus Nerve Stimulation
Central effects of VNS are predominantly believed to be due to activation of vagal afferents, though, as discussed later, immunoregulatory effects of VNS may also affect central nervous system (CNS) physiology and pathophysiology. As most vagal afferents project to the NTS, this nucleus is believed to be an important, initial relay for all effects related to stimulation of vagal afferents ( Figs. 18.1B and 18.2 ). Through the NTS, the vagal afferent system is connected to structures throughout the CNS. In addition to a few vagal afferents passing directly to the dorsal motor nucleus of the vagus and the nucleus ambiguous ( ), the NTS also sends secondary projections to these nuclei, which are involved in regulation of parasympathetic, reflex responses ( ). The NTS importantly connects to several endogenous neuromodulatory nuclei, such as the brainstem noradrenergic locus ceruleus (LC), and indirectly through the LC to the serotonergic raphe nuclei and the dopaminergic ventral tegmental area ( ) ( Fig. 18.1C ). All these nuclei have been reported to be modulated during VNS ( ) in rats, resulting in a regulation of release of neurotransmitters throughout the entire CNS ( Fig. 18.1D and E ). VNS is additionally believed to exert an influence on cholinergic transmission from the basal forebrain nucleus of Meynert ( ), though it is not exactly clear how VNS reaches this nucleus. Finally, the NTS directly connects to several other areas of the brain that include the hypothalamus (both directly and indirectly via the parabrachial nucleus), the thalamus, the hippocampus, and the cortex ( ) ( Figs. 18.1D,E and 18.2 ). Many of the central effects reported in the following sections are thus likely to be a result of a complex interaction between all of these pathways, though some pathways have proved to be more relevant than others.

Anticonvulsant Effects of Vagus Nerve Stimulation
Preclinical evidence regarding anticonvulsant mechanisms of VNS have revealed a potentially important role of noradrenergic transmission through the LC ( Fig. 18.2 ). VNS has been found to increase the tonic activity of LC neurons following just a few hours of stimulation ( ), which is consistent with the reports of increased concentrations of norepinephrine (NE) found in both the limbic system and the cortex induced by VNS ( ). A bilateral lesion of the LC was found to abolish acute anticonvulsant effects of VNS in the corneal stimulation-induced seizure model in rats ( ). Anticonvulsant effects of NE in hippocampal seizure models have previously been attributed to α 2 -adrenergic receptors ( ). Blocking α 2 -adrenergic transmission in the hippocampus, indeed, attenuated anticonvulsant effects of VNS in a hippocampal seizure model in rats ( ). Although direct evidence of an effect of VNS on noradrenergic signaling in patients is lacking, a translation of these experimental findings into human application has been found. The P300 is a cognitive event-related potential, measurable at the scalp and known to be heavily modulated by the noradrenergic system. A recent studied showed a relationship between an effect of VNS on the likelihood for patients with epilepsy to show therapeutic response ( ). The P300 amplitude can be significantly increased when VNS is switched on and off and only in responders to VNS therapy. Prospective trials and pilot studies with tVNS and P300 in patients are ongoing to translate these findings into the identification of biomarkers for response to VNS, an essential issue currently lacking in clinical practice ( ).
A popular hypothesis for anticonvulsant mechanisms of VNS has been desynchronization of brain rhythms, though it is not described how this effect is produced. The hypothesis was sparked from old observations of Zanchetti and colleagues in 1952 that application of VNS in “ecephale isole” preparations of cats under ether anesthesia desynchronized cortical rhythms measured with electroencephalography (EEG) ( ). In 1966 and 1967, Chase and colleagues reproduced these findings but found that low intensities of VNS synchronized while high VNS intensities desynchronized EEG activity ( ). What must be noted is that the lack of digital technology hindered the offline processing of the data according to modern standards, and it is thus difficult to extract exactly what these investigators observed, as their analyses were based on visual inspections of the EEG signals. Recent evidence suggests that VNS decreases EEG power across all frequencies in awake rats ( ). Due to the fact that unit spikes do not contribute to field EEG recorded on the scalp, it is, however, still impossible to make a claim of unit desynchronization based on such recordings. Nevertheless, the idea that VNS desynchronizes brain activity has been amplified through several influential reviews on VNS over the years, though it was only recently that investigators, in fact, have shown increased but decorrelated cortical unit activity during VNS ( ).
Several clinical studies have investigated a potential effect of VNS on EEG parameters in patients with epilepsy, but the outcomes have been highly variable, which is likely due to high variability in the patients studied, small sample sizes, variable VNS treatment durations, and variable analysis methods. Some investigators have reported no effects on EEG ( ); others have noted minor changes in relative power contribution of specific frequency bands ( ) or changes in specific connectivity and synchrony measures ( ). Common to most of these findings is that they have not been reproduced at other research centers. The only consistent finding seems to be the ability of VNS to reduce interictal EEG spiking in the EEG ( ), which is consistent with an anticonvulsant effect. There is, however, insufficient evidence to support desynchronization as an actual anticonvulsant mechanism of VNS.
Other imaging modalities like positron emission tomography (PET), single-photon emission computed tomography (SPECT), and functional magnetic resonance imaging (fMRI) have also been used to investigate mechanisms and structures affected by VNS. What consistently has been found is a change in thalamic activity during VNS, though this may be related to sensory processing as most patients describe a tingling effect in the neck region during VNS. Studies using PET or fMRI to assess structures affected by VNS in epilepsy patients have generally reported an increase in thalamic activity ( ), though decreased thalamic activity generally has been reported in SPECT studies ( ). Several other structures involved include the hippocampus, the amygdala, various areas of the cortex, and the cerebellum, but an effect on the thalamus is the only outcome, which has been reported to be related to therapeutic response ( ).
Antidepressant Mechanisms
The neurobiology of mood disorders remains incompletely understood, but there are several theories based on abundant experimental evidence. Among them is the theory that mood disorders may be caused by an imbalance in the central monoaminergic signaling of the brainstem. Similar to conventional antidepressant medications like serotonin and NE reuptake inhibitors, VNS increases the availability of extracellular NE and serotonin in areas related to regulation of mood, such as the limbic system and the prefrontal cortex ( ) ( Fig. 18.2 ). In a recent study in rats, lesioning of the noradrenergic system was found to attenuate the antidepressant effects of VNS, supporting an important role of the LC–noradrenergic system in the antidepressant effects of VNS ( ). Additionally, PET and SPECT imaging studies in depressed patients have shown that the application of VNS leads to a change in activity in several of the aforementioned relevant areas for mood disorders, though it is unknown whether this is a consequence of altered monoaminergic neurotransmission ( ).
VNS in rats is further found to facilitate neurogenesis in the dentate gyrus, one of the few places in the brain where the formation of new neurons takes place throughout life ( ). This is consistent with findings of increased hippocampal expression of neurotrophic growth factors such as basic fibroblast growth factor (bFGF) and brain-derived neurotrophic growth factor (BDNF) ( ). Previous studies have revealed a loss of hippocampal neurons in animal models of depression ( ) and hippocampal volume loss visualized on MRI in depressed patients ( ). Because remission from depressive symptoms is associated with normalization of these neurobiological features ( ), this has been discussed as a likely neurobiological antidepressant mechanism of VNS ( ).
Analgesic Mechanisms
A series of rat and primate studies conducted in the late 1970s and 1980s demonstrated that analgesia is potentially mediated by vagal afferents that inhibit spinal nociceptive reflexes and transmission. Application of electrical stimulation to the proximal end of the transected vagus has been found to inhibit the response to graded noxious skin heating of second-order nociceptive neurons at all levels of the spinal dorsal horn and the trigeminal nucleus ( ). Even third-order thalamic neurons have been reported to be inhibited by VNS ( ). An anesthetic block to the NTS was found to eliminate this effect ( ), while either electrical or glutamatergic stimulation mimicked antinociceptive effects of VNS ( ). The NTS is believed to modulate the activity of brainstem nuclei, which are a part of a well-characterized descending inhibitory system, formed primarily via projections to the dorsal horn of the spinal cord and trigeminal nucleus. These nuclei include the periaqueductal gray, the nucleus raphe magnus, and the LC ( ). Activation of these structures during VNS is believed to result in the release of several substances at the level of the spinal cord, including NE and serotonin. Administration of either α-adrenoceptor or serotonergic receptor antagonists attenuates the antinociceptive effects of VNS ( ). The observation that administration of opioid receptor antagonists similarly attenuates the antinociceptive effects of VNS has further facilitated the idea of opioidergic involvement ( ). In patient studies, VNS modulates brain areas involved in the affective processing of pain, including the anterior cingulate cortex and the insula ( ). Though evidence clearly supports an analgesic effect of VNS, clinical evidence is currently limited to either open-label trials in headache syndromes or fibromyalgia with variable response rates observed ( ).
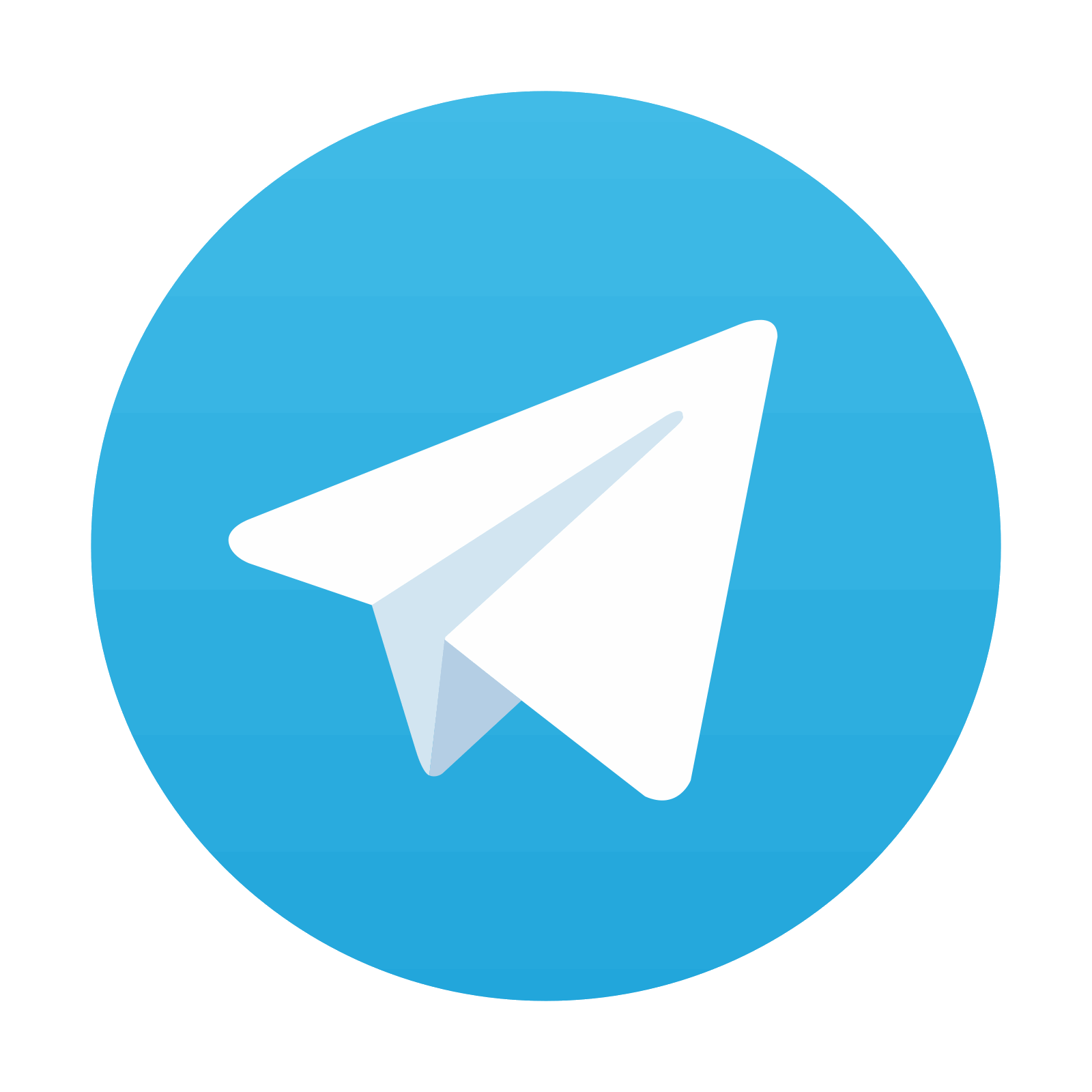
Stay updated, free articles. Join our Telegram channel
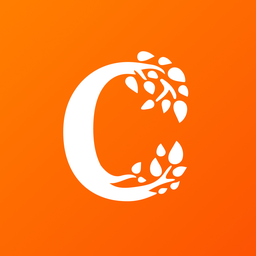
Full access? Get Clinical Tree
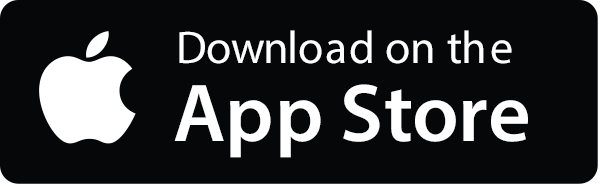
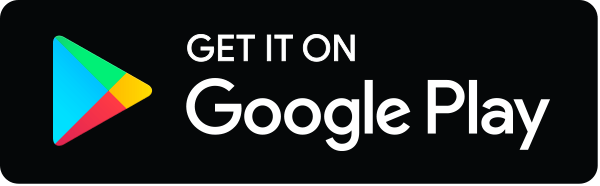