Visual Pathways
Anatomy of the Visual System
The Retina
The retina extends anteroposteriorly from the ora serrata to the optic disc, which corresponds to the attachment of the optic nerve, slightly nasal to the posterior pole of the eyeball. Approximately at the posterior pole of the globe is the macula, a circular area of the retina that appears yellow when viewed with the ophthalmoscope. Each retina can be divided into four quadrants by a vertical and a horizontal meridian intersecting at the macula (Fig. 7.1). The horizontal meridian separates the retina into superior and inferior portions. The vertical meridian separates the nasal (medial) retina from the temporal (lateral) retina.
The first neuronal elements in the visual system are located deep in the retina, separated from the choroid by the retinal pigment epithelium. These elements, the rods and cones, contain pigments that, reacting to visible light, produce electrical activity. This activity is conveyed to the more superficially located ganglion cells by short bipolar cells and by horizontally disposed amacrine cells (Fig. 7.2). The ganglion cells send their axons predominantly to the lateral geniculate body or to the superior colliculus.
The photoreceptors, rods and cones, are oriented toward the pupillary opening rather than toward the center of the globe. The pigment of the rods is a glycoprotein called rhodopsin, which reacts to light within the visible wavelength, from 400 to 800 nm. Approximately 100 million rods are unevenly distributed throughout the retina. They become more tightly packed in the fundus of the globe but are absent from the optic disc (blind spot) and from the macula.
There are three different types of cones that react maximally to red, green, or blue light. The retina contains approximately 7 million cones, 100,000 of which are concentrated in the macular region. In the center of the macula, there is a small region (the foveola, measuring 0.35 mm across) that is devoid of vessels and neural elements other than the tightly packed cones. Visual discrimination is greatest here, where light can reach the photoreceptors avoiding the layers present in the rest of the retina.
An estimated 1.2 million ganglion cells populate the inner aspect of the retina. Their receptive fields become smaller in the region of the posterior pole of the globe, where ganglion cells are much more numerous than in the periphery and the cones have one-to-one connections with their own ganglion cells. By contrast, in the periphery, receptive fields overlap extensively. This anatomic arrangement may explain the relative sparing of the peripheral vision with lesions that affect the ganglion cells preferentially.
Morphologically different classes of retinal ganglion cells (M cells and P cells) project to different divisions of the lateral geniculate nucleus, which, in turn, project to the visual cortex in a segregated distribution. M cells make up approximately 10% of the retinal ganglion cells and are engaged with “where” the target of regard is in space. They are concerned with depth perception or stereopsis, are color ignorant, and have high contrast sensitivity, low spatial resolution, and fast temporal resolution. Retinal M cells project to magnocellular neurons in layers 1 and 2 of the lateral geniculate nucleus, which in turn project to 4C a neurons in cortical area 17. The 4C a neurons project to 4 b cortical area 17 neurons, which in turn project to cortical area MT (see Chapter 8). P cells only slightly outnumber M cells in the peripheral retina, whereas the macula is composed predominantly of P cells, which are concerned with “what is being seen” (they have color opponency, low contrast sensitivity, and high spatial resolution). P cells make up approximately 90% of retinal ganglion cells and project to parvocellular neurons in layers 3, 4, 5, and 6 of the lateral geniculate nucleus, which project to 4C b neurons in cortical area 17. The 4C b neurons project to layers 2 and 3 of cortical area 17 which, in turn, project to cortical area 18, which then sends fibers to areas V3 and V4 [48].
Certain pathologic processes may preferentially affect M cells or P cells. In Alzheimer’s disease, for example, there is a predominant loss of M cells in the retina, resulting in difficulty with determining motion and depth and inaccurate fast eye movements (saccades) with preserved acuity and color vision [168]. In optic neuritis (ON), more P than M ganglion cells are lost, which may explain contrast sensitivity abnormalities, central scotomata, and color vision impairment [198].
The axons of the ganglion cells constitute the innermost layer of the retina, which is separated from the vitreous by a thin basement membrane. The position of the axons in the nerve fiber layer depends on their origin in the retina. As the axons converge toward the optic disc, the ganglion cells closer to the disc send their axons through the whole thickness of the nerve fiber layer. Therefore, the more peripherally generated axons are deeper in this layer, whereas the ones originating centripetally rest nearer to the vitreous (Fig. 7.2.)
FIG. 7.1. Retinal nerve fiber layer and arteries. Note the temporal raphe formed by the fibers from the superior and inferior retina.
FIG. 7.2. Diagrammatic representation of the retinal layers, disposition of fibers in the nerve fiber layer and optic nerve, and visual field defects caused by retinal or optic nerve lesions. The vertical bars (a, b, c) represent partial (a) to complete (c) retinal lesions; the corresponding field defects are depicted underneath. Retinal lesions affecting the nerve fiber layer have an arcuate shape with the base located peripherally and, in temporal retinal lesions, in the horizontal meridian. Compare with Figure 7.1.
FIG. 7.3. Schematic horizontal section at the level of the lateral geniculate bodies, depicting the optic pathways. The right hemifield has been shaded, and fibers from the corresponding retina have been traced.
Nerve fibers nasal to the optic disc and those originating in the nasal side of the macula (papillomacular bundle) take a straight course as they converge into the optic disc (Fig. 7.1). The remaining fibers arch around the papillomacular bundle, adopting a disposition that has a bearing on the visual field defects that are secondary to retinal and optic nerve lesions. Fibers from the superior half of the temporal aspect of the macula arch superiorly and then down toward the disc. Fibers from the inferior half of the temporal aspect of the macula arch inferiorly and then ascend to reach the disc. Fibers from the temporal retina, particularly those closer to the horizontal meridian, follow a similar course. Therefore, between the nerve fibers from the superior temporal retina and those from the inferior temporal retina a raphe is formed, located in the horizontal meridian (Fig. 7.1).
The axons of the ganglion cells on the temporal side of a vertical line drawn through the fovea project to the ipsilateral lateral geniculate body, whereas the ones from the nasal side cross at the optic chiasm (Fig. 7.3). However, this separation is not sharp. The neurons subserving the macular region and a vertical strip of approximately 1 degree, centered in the fovea, project to either lateral geniculate body.
The Optic Nerves and Optic Chiasm
Each optic nerve is approximately 50 mm long and has four portions from the globe to the chiasm (Fig. 7.4).
1. Intraocular Portion. In this portion, also called the optic nerve head (1 mm long), the axons become myelinated (central type of myelin). The funduscopic appearance of the optic nerve depends on the angle of the nerve head to the eye. When the angle between the nerve and the sclera is <90 degrees, a rim or crescent of choroid or sclera may be seen on the flat temporal side of the disc, whereas the nasal edge appears elevated.
2. Intraorbital Portion. This (section 25 mm long) is shaped like an elongated S to allow mobility within the orbit. Here the optic nerve is surrounded by fat contained in the cone formed by the ocular muscles. The apex of this cone (which is open to the optic foramen and the superior orbital fissure) is directed posteriorly and slightly displaced nasosuperiorly in the orbit (Fig. 7.4). In addition to the ophthalmic artery, the ciliary ganglion and nerves, and the nerves to the extraocular muscles are in close relation to the optic nerve here.
FIG. 7.4. Superolateral view of the contents of the sella and cranial nerves in the cavernous sinus.
3. Intracanalicular Portion. This portion (approximately 9 mm long) is the part of the nerve that travels the optic canal. Each optic canal is oriented posterosuperomedially, at an angle that approximates 45 degrees to the sagittal and horizontal planes. The ophthalmic artery and some filaments of the sympathetic carotid plexus accompany the optic nerve within the optic canal.
4. Intracranial Portion. This part (approximately 4–16 mm long, depending on the position of the chiasm) stretches between the proximal opening of the optic canal and the chiasm (Fig. 7.4). Each optic nerve lies above the respective carotid artery as this vessel exits from the cavernous sinus and gives off the ophthalmic artery. Inferomedially, the optic nerve lies over the bony roof of the sphenoid sinus, which can be quite thin, and over the contents of the sella turcica when the chiasm is posteriorly placed. Superior to each optic nerve is the horizontal portion of the anterior cerebral artery, which is overlaid by the gyrus rectus of the frontal lobe, the olfactory tract, and the anterior perforated substance (Fig. 7.5). The anterior communicating artery is superior to the optic nerves or to the optic chiasm.
Proximal to the angled optic canal, the optic nerves maintain a 45-degree angle to the horizontal plane, and the chiasm is similarly tilted over the sella turcica, with the suprasellar cistern lying between them. The relation between the chiasm and the sella varies between individuals. In brachycephalic heads the chiasm tends to be more anterior and dorsal than in dolichocephalic heads. Autopsy studies have shown that in approximately 5% of individuals the chiasm overlies the anterior margin of the sella (prefixed chiasm), in 12% it lies over the diaphragma sellae, in 79% it is above the dorsum sellae, and in 4% it projects behind the dorsum sellae (postfixed chiasm). The chiasm is located below the suprachiasmatic recess of the third ventricle in close proximity to the hypothalamus. Above the chiasm are the lamina terminalis and the anterior commissure. Immediately posterior to it, the pituitary stalk runs an anteroinferior course. The optic tracts originate from the posterolateral corners of the chiasm (Fig. 7.5).
Nerve fibers in the optic nerve follow a topical arrangement similar to that found in the retina (Fig. 7.6). Superior retinal fibers run superiorly in the optic nerve, inferior fibers are below, and those from the temporal and nasal retina run in the corresponding parts of the optic nerve. In the proximal portion of the nerve, near the globe, the macular fibers occupy a wedge-shaped sector just temporal to the central vessels (Fig. 7.6). More distally, they shift toward the core of the nerve.
FIG. 7.5. Inferior aspect of the brain showing some relationships of the optic nerves, chiasm, and optic tracts.
At the chiasm, more than half of the fibers (those originating in ganglion cells of the nasal retina) cross to reach the contralateral optic tract (Fig. 7.3). The ratio of crossed to uncrossed fibers is approximately 53:47. Fibers from the inferior part of the nasal retina are ventral in the chiasm and loop into the proximal portion of the contralateral optic nerve (Wilbrand’s knee) before reaching the lateral aspect of the optic tract (Fig. 7.7). Those from the superior nasal retina remain dorsal in the chiasm and become medial in the optic tract.
The anatomic existence of Wilbrand’s knee has come into question. Wilbrand was restricted to examining human subjects who had undergone enucleation. In the enucleated eye, the nerve fibers atrophied and became distinct from the nerve fibers of the normal eye as seen on myelin staining. Horton, utilizing axon labeling techniques in nonenucleated monkeys, was unable to demonstrate crossing fibers looping into the contralateral optic nerve (Wilbrand’s knee) [72]. In one monkey that had undergone enucleation 4 years previously, however, nerve fiber topography similar to that described by Wilbrand was found. Horton hypothesized that Wilbrand’s knee may be an artifact of enucleation caused by atrophy of the optic nerve and not a normal anatomic finding. However, the concept of Wilbrand’s knee is still clinically useful (see subsequent text).
FIG. 7.6. Disposition of the ganglion cell axons in a cross-section of the optic nerve. A: Distal portion, near the globe. B: Proximal portion, where the macular fibers have shifted to the core of the nerve.
FIG. 7.7. Crossing of nasal fibers in the optic chiasm. Fibers from the inferior retina make a forward loop into the opposite optic nerve (Wilbrand’s knee). The existence of Wilbrand’s knee has recently been questioned (see text). (Figure modified from Hoyt WF, Luis O. Visual fiber anatomy in the infrageniculate pathway of the primate. Uncrossed and crossed retinal quadrant fiber projections studied with Nauta silver stain. Arch Ophthalmol 1962;68:94–138.)
Uncrossed fibers, originating from the temporal retina, maintain their dorsal or ventral position in the chiasm. The macular fibers, which constitute a large proportion of the total number of chiasmal fibers, are also crossed and uncrossed. However, the separation between temporal and nasal ganglion cells is not sharp. Crossed and uncrossed fibers originate in both nasal and temporal sides of the macula. In the optic tract, the macular fibers occupy a dorsal position.
The Optic Tracts and Lateral Geniculate Bodies
The optic tracts extend from the dorsolateral corners of the chiasm to the lateral geniculate bodies. From the chiasm the tracts run posterolaterally, limiting the hypothalamus to a triangular space; they then sweep around the cerebral peduncles, and, as soon as they cross them, reach the lateral geniculate bodies in the posterior part of the ventral aspect of the thalami (Fig. 7.3). Several large vessels are located below the optic tracts. The posterior communicating artery crosses their distal portion in the suprasellar cistern. In the perimesencephalic cistern, the posterior cerebral artery and the basilar vein of Rosenthal are apposed to the tracts. Inferolaterally, the uncus of the temporal lobe covers the proximal portion of each tract (Fig. 7.5).
The lateral geniculate body, a thalamic nucleus, provides a relay station for all the axons of the retinal ganglion cells subserving vision. Neurons from the lateral geniculate body project, by way of the optic radiations, to the pericalcarine cortex of the occipital lobe, which is the primary cortical area for vision (Fig. 7.3). The lateral geniculate body is in the roof of the perimesencephalic cistern (cisterna ambiens), just medial to the hippocampal gyrus of the temporal lobe. Anteriorly, the lateral geniculate body receives the optic tract and sends out the ventral optic radiations, which lie in a close association with the posterior limb of the internal capsule. Dorsolaterally, the lateral geniculate body is covered by the optic radiations. Dorsomedial to the lateral geniculate body, the auditory radiations, originating from the medial geniculate body, pass on their way to the transverse temporal gyrus of Heschl, where the primary auditory cortex is located. Superomedial to the lateral geniculate body is the pulvinar of the thalamus.
Shaped on midsection like Napoleon’s hat, with its concave aspect (hilus) facing inferoposteromedially, the lateral geniculate body has a deep brown color with stripes (striae) of white matter that are visible to the naked eye [76]. The geniculate neurons, as numerous as the fibers in the optic tract, are disposed in six laminae, numbered from I to VI, beginning from the hilus of the nucleus. Layers I, IV, and VI serve the contralateral eye, whereas II, III, and V are connected with the ipsilateral eye. These six laminae are clearly distinguished in the center of the lateral geniculate body, where the macular region of the retina is represented, but only one or two are present in the peripheral part of the nucleus, which receives axons from ganglion cells in the peripheral retina.
The postchiasmal shift in the position of the fibers (the superior retinal fibers become superomedial and the inferior fibers become inferolateral; Fig. 7.7) persists in the synaptic areas of the lateral geniculate body. This shift is straightened out in the optic radiations, where again the superior fibers correspond to the superior retina and those below to the inferior retina. A similar representation is found in the calcarine cortex.
The Optic Radiations
The optic radiations sweep posteriorly around the lateral aspect of the posterior portion of the lateral ventricles (Fig. 7.8), forming the external sagittal stratum, which is separated from the ventricle by the internal sagittal stratum, made up of occipitomesencephalic fibers. Three bundles can be distinguished in the radiations: (a) the upper bundle, originating in the medial part of the lateral geniculate body and corresponding to the superior retina, which courses through the deep parietal white matter and ends in the superior lip of the calcarine fissure; (b) the central bundle, originating from the medial part of the nucleus and serving the macular region, which travels through the posterotemporal and occipital white matter and ends in the posterior part of the calcarine fissure, on both lips; and (c) the lower bundle, originating from the lateral part of the nucleus and corresponding to the lower retina, which sweeps first anteriorly and then posteriorly around the temporal horn of the lateral ventricle (Fig. 7.8), terminating in the lower lip of the calcarine fissure. As they sweep lateral to the ventricle (Meyer’s loop), the lower radiations reach a point located approximately 5 cm behind the tip of the temporal lobe.
In the anterior part of the radiations, fibers corresponding to adjacent retinal units are spatially separated and the macular fibers, instead of being interposed between fibers from the superior and inferior peripheral retina, run medial to them. Also, fibers from either eye seem to have a similar anterior extent in Meyer’s loop, with those from the contralateral eye lying medial to the ones coming from the ipsilateral eye.
FIG. 7.8. Lateral view of the brain showing the arrangement of the optic radiations in the parietal and temporal lobes, lateral to the ventricular system.
FIG. 7.9. Schematic diagram showing arrangement of V1, V2, and V3 along the medial and posterior occipital surface. Most of V1 is buried within the calcarine fissure. (From Horton JC, Hoyt WF. The representation of the visual field in human striate cortex. A revision of the classic Holmes’ map. Arch Ophthalmol 1991;109:816. Copyright 1991, American Medical Association. Reprinted with permission.)
The Visual Cortex and Visual Association Areas
Cortical area 17 of Brodmann, located along the superior and inferior lips of the calcarine fissure in the medial aspect of the occipital lobe, receives the axons from the neurons of the lateral geniculate body and represents the first link in the cortical processing of visual information (primary visual cortex, see Chapter 20). The primary visual cortex actually extends farther than the posterior extent of the calcarine fissure, spreading for approximately 1 cm around the posterolateral aspect of the occipital pole. On cross-section of the cortex, a white matter stria (stria Gennari) can be seen with the naked eye. This characteristic feature has won the term striate cortex for area 17. The line of Gennari corresponds to a thick band of white matter in layer IV of the cortex, which is devoid at this point of pyramidal cells but is very rich in granular cells.
Each occipital lobe receives projections from the nasal half of the opposite eye and from the temporal half of the ipsilateral retina. More simply, it receives projections from the two halves of the retinas on the same side as the occipital lobe (Fig. 7.3). This unilateral representation includes the macular region. The superior and inferior retinal projections extend to the superior and inferior lips of the calcarine fissure, respectively. Finally, the macular retina is represented in the posterior pole of the calcarine cortex, whereas the more peripheral retina is more anteriorly represented [180]. The foveal representation is located at the occipital pole, where the striate cortex usually extends approximately 1 cm onto the lateral convexity of the occipital lobe. The extreme periphery of the visual field is represented anteriorly at the junction of the calcarine and parieto-occipital fissures. The central 10 to 15 degrees of vision fill most of the total surface area of the occipital cortex (as much as 50%–60%) (Figs. 7.9 and 7.10) [75,125].
Vascular Supply of the Visual Pathways
The vascular supply of the retina is derived from the ophthalmic artery, which branches from the carotid artery shortly after this vessel exits from the cavernous sinus. At the optic canal, the ophthalmic artery lies below and lateral to the nerve. At a point 5 to 15 mm from the globe, it gives off the central retinal artery, which pierces the optic nerve and courses forward in its core, to divide into a superior and an inferior branch at the optic disc (Fig. 7.1). Second-order nasal and temporal branches supply the nerve fiber layer and the inner layers of the retina (including ganglion cells). From the anatomic arrangement of these vessels, it follows that the complete occlusion of the central retinal artery results in global retinal ischemia, except when the macular area is supplied by cilioretinal arteries, and occlusion of one of its branches causes superior or inferior retinal ischemia. The consequence of such a lesion is an inferior or superior altitudinal field defect (Fig. 7.2). Infarction in the territory of the central retinal artery may be caused by emboli, thrombi, hypercoagulable states, migraine, and arteritis (e.g., giant cell arteritis).
FIG. 7.10. A: Artificially flattened map showing retinotopic organization of V1 (stippled area), V2 (small triangles), and V3 (hatched) in the left occipital lobe. B: Right visual field coordinates corresponding to map in (A). More than half of the visual cortex is devoted to processing the central 10 degrees of vision. (From Horton JC, Hoyt WF. The representation of the visual field in human striate cortex. A revision of the classic Holmes’ map. Arch Ophthalmol 1991;109:816. Copyright.)
In addition to the central retinal artery, the ophthalmic artery gives off dural branches (anterior falcine and recurrent meningeal arteries), orbital branches, and several posterior ciliary arteries. The posterior ciliary arteries form a rich anastomotic circle on the posterior aspect of the globe near the optic nerve and supply some sectors of the optic disc, the outer layers of the retina (including photoreceptors), and the choroid. In about half of the population, the region of the macula and papillomacular bundle receives its vascular supply from one or more cilioretinal arteries, which are branches of the posterior ciliary arteries (Fig. 7.1). This explains the sparing of central vision that occurs in some individuals despite central retinal artery occlusion (CRAO) with global retinal ischemia. Unlike ischemia in the territory of the central retinal artery, ischemia in the territory of the posterior ciliary arteries is seldom related to emboli but is usually caused by either atherosclerotic disease or hypotension (nonarteritic anterior ischemic optic neuropathy [AION]) or a vasculitis (arteritic ischemic optic neuropathy [ION] due to temporal or giant cell arteritis). However, field defects resulting from lesions in the territory of the posterior ciliary arteries are also altitudinal, and the differentiation of lesions in either territory rests on the ophthalmoscopic findings. Edema of the retina is obvious in the acute stages of retinal infarction owing to CRAO, but the retina may appear normal or show axonal swellings (cotton wool spots) in a segmental distribution in the presence of ischemia in the territory of the posterior ciliary arteries.
The distal part of the optic nerve (near the globe) is supplied by small branches of the ophthalmic artery and, as it approaches the chiasm, by thin vessels from the carotid and anterior cerebral arteries. Similarly, thin vessels originating in the region of the anterior communicating artery supply the dorsal aspect of the chiasm, whereas the inferior aspect receives arterioles from the carotid, posterior communicating, and posterior cerebral arteries. The latter two vessels also supply the optic tract, which in addition is fed by the anterior choroidal artery, a branch of the internal carotid. The lateral geniculate body receives a dual supply, from the anterior choroidal artery laterally and from the lateral posterior choroidal artery medially. The upper (parietal) portion of the optic radiations is supplied by branches of the middle cerebral artery, whereas the lower part receives branches from the posterior cerebral artery. The posterior cerebral artery, particularly its calcarine branch running in the calcarine fissure, supplies the primary visual cortex. Anastomotic branches from the middle cerebral artery (generally the angular or posterior temporal arteries) also play an important role in the vascular supply of the occipital pole, in which the macular region is represented.
Localization of Lesions in the Optic Pathways
The long course of the visual pathways along the base of the brain and their relative simplicity render them a very useful tool in lesion localization. Various techniques of neuro-ophthalmologic testing are reviewed by Glaser [48]. Detailed quantitative testing allows the following:
1. The detection of subtle deficits that may escape detection by bedside maneuvers
2. Better definition of abnormalities, such as the exact shape of a field defect, which may be important in lesion localization
3. The quantification of the extent and intensity of a deficit, which are very useful data when judging the evolution of a disease process
However, detailed testing requires equipment that is unavailable at the bedside and a degree of active cooperation that is often lacking in patients with brain disorders.
Lesions in the visual system may cause impaired visual perception or objective deficits. Impaired visual perception may include (a) poor discrimination of fine details of high contrast (visual acuity), which results in difficulty with tasks such as reading a printed page; (b) impaired color recognition; (c) impaired discrimination of objects that have little contrast with the background (contrast discrimination); and (d) visual field defects, the pattern of which is often the most helpful clue to lesion localization. Objectively, retinal changes caused by retinal or more proximal lesions can be seen with the ophthalmoscope, and an impaired pupillary response to light may betray a lesion in the afferent arc of this reflex.
Changes in Visual Perception
VISUAL ACUITY
Visual acuity, the capacity for visual discrimination of fine details of high contrast, such as small black letters on a white page, reflects the function of the macular region. A subnormal value of acuity indicates a fault in the visual system (e.g., optical faults, retinal lesions, or visual pathway lesions), faulty foveation (i.e., an eye motility defect), or poor cooperation, singly or in combination [42]. It remains unimpaired by unilateral lesions dorsal to the optic chiasm [42]. In practice, visual acuity is most often impaired by changes in the shape of the globe and in the refractory characteristics of the transparent media of the eye. Patients with these refractory defects regain a much better acuity when looking through a pinhole (pinhole test) because this maneuver restricts vision to the central beam of light, which is undisturbed by abnormal ocular distances or transparent media. At the bedside, visual acuity can be tested by asking the patient to read a “near card” with the Snellen optotypes printed on it. The card should be well-illuminated and held 14 inches in front of the patient’s eyes.
Once refractory defects have been excluded, it can be accepted that changes in visual acuity are secondary to lesions in the macular region or its projection. The macula is the only part of the retina that has high visual acuity. Virtually, all compressive and most noncompressive lesions of the optic nerve cause a drop in visual acuity, often even before a field defect can be detected. Medial chiasmal lesions behave in a similar manner. Lateral chiasmatic lesions tend to impair visual acuity in the ipsilateral eye only. From these findings and from the sparing of visual acuity that occurs with retrochiasmatic lesions, Frisen postulated that acuity will remain normal if either the crossing or the noncrossing set of nerve fibers from the fovea remains intact [42]. Both sets of fibers are often affected with medial chiasmatic lesions. Unilateral lesions of the optic tract, lateral geniculate body, visual radiations, or striate cortex do not impair visual acuity. When the retrochiasmal pathways are affected bilaterally, visual acuity fails to the same degree in both eyes.
CONTRAST SENSITIVITY
Contrast sensitivity testing may detect more subtle impairments in the function of the macula, optic nerve, and chiasm than visual acuity testing [18,103]. For instance, visual acuity may become normal after an acute ON, yet the patient may complain of “dimness” or “fuzzy vision” in that eye. This patient’s ability to perceive a series of bars that have very little contrast from the background will probably be abnormal. Impaired contrast sensitivity probably has localizing significance that is similar to that of impaired visual acuity, but it has been studied less thoroughly.
PERCEPTION OF COLOR
Color perception is often degraded in areas of the visual fields that correspond to a partial field defect. For instance, a scotoma for blue or for red may be demonstrated when vision for white targets is still good. In confrontation testing of the visual fields, one of the most useful techniques is to ask the patient which one of two identically bright red objects is more red, because a desaturation for red is often caused by lesions of the visual pathways. A color sample that appears red to the healthy eye appears more yellowish to the defective eye and passes from orange to yellow to colorless as disease severity increases. Impairment of color vision may also be detected by asking the patient to read numbers composed of an assembly of dots of different colors embedded in a background of differently colored dots (Ishihara or Hardy–Rand–Rittler pseudoisochromatic color plates). Color-blind patients cannot perform this task, which mainly reflects macular function. Because optic nerve and chiasmatic lesions often affect the macular fibers, monocular reading of the Ishihara or similar plates may be defective on the side of the lesion. However, Ishihara plates generally have poor sensitivity for acquired dyschromatopsia. It should also be noted that the interpretation of pseudoisochromatic color plates requires that the patient is able to “put the dots together” to make a visual whole. Therefore, patients with simultanagnosia (see Chapter 20) due to bilateral occipitoparietal damage (e.g., in the “posterior” form of Alzheimer’s disease) may have difficulty in identifying the images on the plates despite adequate visual acuity and the ability to name all the colors in the plates correctly [17].
Color vision loss usually parallels visual acuity loss (e.g., in ION), but in ON color vision loss may be much worse. In ON, chromatic sensitivity is more severely impaired than luminance sensitivity [132]. Another exception to the general rule of color vision failure paralleling visual acuity impairment is that color vision does not depend equally on perfect foveation and a well-focused retinal image, so that patients with nystagmus and anisometropia usually have normal color vision unless acuity is severely impaired. Acuity may also be normal with acquired achromatopsia due to cerebral cortical lesions [32,157].
Congenital color vision defects are much more common in men than women, with deficits mainly of red and green hues. Acquired color vision defects cluster primarily in the blue–purple or blue–green hues [131]. Therefore, bluish–purple objects may have superior sensitivity over red targets in assessing acquired dyschromatopsia. In general, patients with primary optic nerve disease frequently show a tendency for hue discrimination difficulties between reds and greens, whereas patients with primary retinochoroidal disorders more frequently show evidence of hue discrimination difficulties between blues and yellows (Köllner’s rule) [63]. However, Köllner’s rule has numerous exceptions (e.g., primary open angle glaucoma is an optic nerve disease characterized by blue–yellow deficits).
Another way of revealing impaired color processing involves the flight-of-colors phenomenon, which consists of a succession of color impressions that normally follows shining a bright light into the eye. This phenomenon is absent or reduced in duration with acquired dyschromatopsia [39].
Impairment of color perception also occurs with lesions in the posterior visual pathways. A visual field defect for red may betray the presence of a lesion when the fields for white stimuli are full. Patients with bilateral lesions of the inferomedial occipital region often have color blindness with normal visual acuity [32,157].
VISUAL FIELDS
The shape and distribution of visual field loss closely reflects the site of the lesion (Fig. 7.11). Therefore, careful plotting of the visual fields is most helpful in the localization of lesions of the visual pathways when examining a cooperative patient [42]. In patients with a markedly reduced attention span or other disturbances in alertness or mentation, the gross extent of the visual fields can be estimated from the patient’s response to moving objects in different quadrants. A moving object strongly induces the patient to look at it. However, small field defects are missed with confrontation techniques [184]. In cooperative patients, visual field testing with the tangent (Bjerrum) screen or static or kinetic perimetry provides a detailed map of the visual fields. Testing of the central 20 to 30 degrees of vision is most important because very few disease processes affect the peripheral fields alone; exceptions to this are the tapetoretinal degenerations and retinal detachment (both diagnosed by ophthalmoscopic exam) and anterior visual cortex lesions [42].
Adequate visual field testing requires patient cooperation and a skilled examiner. Shadowing facial contours (e.g., the eyebrows and nose), ptosis, disorders of eye motility, blinks, pupillary size, eyelashes, and spectacle rims must all be taken into account when interpreting the visual fields [42]. Ametropia, presbyopia, or both may affect the fields. For example, uncorrected astigmatism may cause an upper temporal depression suggesting a chiasmatic lesion; however, unlike a true chiasmatic defect, this defect does not respect the vertical meridian and spares central fixation [42]. Spherical ametropia may cause generalized field depression and occasionally an upper temporal depression, which may run under the blind spot (baring of the blind spot) due to local ametropia or local deviation from the normal retinal curvature [42].
By convention, in representing the visual fields, the field for the left eye is represented to the left of the field for the right eye (Fig. 7.11). Therefore, the nasal retina of the left eye “sees” the temporal field of the left eye. This terminology explains why a chiasmatic lesion that destroys the nasal fibers from both retinas causes a bitemporal hemianopia. Similarly, a macular lesion yields a central defect, whereas a lesion in the nasosuperior retina of the right eye results in a field defect in the temporoinferior portion of the visual field corresponding to the right eye.
FIG. 7.11. Visual field defects with chiasmatic and retrochiasmatic lesions. Visual fields from both eyes are usually abnormal. There is greater similarity between the field defects in each eye (congruity) with more posteriorly located lesions.
Any localized area of poor vision surrounded by areas of normal vision is termed a scotoma. The blind spot, the projection of the optic nerve in the visual field, is a physiologic scotoma that cannot be perceived because it lacks representation in the brain. Angioscotomata, the shadow images of the superficial retinal vessels on the underlying retina, is another type of physiologic scotoma that may be noted under certain circumstances. Absolute defects involving the outer limits of the visual field are called contractions, whereas depressions are smoothly tapering but not absolute deficits in the field [42].
Types of Visual Field Defects
A central defect occupies the position of the macula (Fig. 7.2). A cecocentral defect affects the area of the macula and of the papillomacular bundle. Nerve fiber bundle defects are field abnormalities in which at least part of the border coincides with the course of the retinal nerve fiber layer [42]. Peripheral nerve fiber bundle defects in the nasal field tend to have an arcuate shape when they are secondary to retinal or optic nerve disease, as they often are. The field defect takes this arcuate shape because of the disposition of the fiber layer in the retina and in the optic nerve (Fig. 7.2). Arcuate field defects may occur with glaucoma, AION, drusen of the disc, and congenital optic pits. Small deep retinal lesions result in a discrete defect localized to the point of the lesion, because the fiber layer remains unaltered. Larger lesions affect the superficial fiber layer and therefore give rise to a fan-shaped arcuate defect, with its tip pointing to the lesion and its base fanning peripherally and toward the nasal horizontal meridian. Nerve fiber bundle defects occur most commonly with lesions in the optic nerve head, where the tip of the defect reaches to the blind spot (Fig. 7.2), but may also occur with branch retinal artery or vein occlusion and with juxtapapillary inflammation. Defects in the temporal field lateral to the blind spot have the appearance of a sector rather than an arcuate shape. Visual defects in the temporal field do not “respect” the horizontal meridian because neither the blood supply nor the fibers of the nasal retina are arranged along a horizontal raphe (as opposed to temporal retinal fibers). The straight course of the retinal fibers of the nasal retina toward the nerve head explains this sector configuration (Fig. 7.1).
Enlargement of the blind spot is often noted with any process causing disc swelling (e.g., increased intracranial pressure); however, any peripapillary retinal disorder (e.g., the peripapillary conus or crescent seen with aging, myopia, or glaucoma and congenital optic nerve pit) may also enlarge the blind spot. These abnormalities are usually observed on ophthalmoscopic examination.
Occasionally a field defect has the appearance of a ring, with preserved vision central and peripheral to the scotoma. Usually the center coincides with the fovea. Annular or ring scotomas may occur with retinopathies or optic neuropathies. Retinitis pigmentosa often results in a large midperipheral ring scotoma. Cancer-associated retinopathy (CAR) syndrome may also cause a ring scotoma, often associated with funduscopic evidence of arteriolar narrowing and optic atrophy [29]. Ring scotomas of small diameter may occur with macular lesions, especially associated with a “bull’s-eye” appearance on ophthalmoscopy (e.g., chloroquine retinopathy [64]) or with the retinal disorder fundus flavimaculatus [156]. Annular or ring scotomas may also occur with retinitis, choroiditis, retinal migraine, and myopia. Paracentral and arcuate scotomas are characteristic of glaucoma; fusion of superior and inferior arcuate defects gives rise to ring scotomata. These ring-shaped defects have a characteristic horizontal or nasal step (Fig. 7.2), which distinguishes them from lesions located more distally in the visual pathways. Physiologic ring scotomas may be caused by corrective lenses, the prismatic effects of strongly curved correcting lenses, and the shallow ring scotoma surrounding the blind spot [42].
When only central vision is intact, the visual field is said to be narrowed, and the patient has funnel vision, not to be confused with tunnel vision, a field defect characteristic of hysteria or malingering. This latter field defect can easily be mapped onto a tangent screen by plotting the fields with the patient seated 1 and 2 m from the screen (the target size is doubled at 2 m) or can be detected with confrontation methods. Logically, with an organic field defect, the field projected at 2 m is larger than the field plotted at 1 m (funnel vision). Identical fields are obtained when the constriction of the field is not due to a lesion of the visual system. Constricted visual fields with retained acuity may be due to glaucoma, retinitis pigmentosa, CAR, hyaline bodies of the disc, postpapilledema optic atrophy, bilateral occipital infarcts with macular sparing, and feigned visual loss.
Hemianopia is a field defect that encompasses roughly half of the field, with a fairly sharp cutoff at the vertical or horizontal meridian (Fig. 7.11) [178]. Vertical hemianopia can be nasal or temporal. Horizontal or “altitudinal” hemianopia can be superior or inferior. When only one-fourth of the field is affected, the resulting deficit is called quadrantanopia.
Bilateral field defects are said to be homonymous when they are similarly located in both visual fields. They are congruous when there is a point-to-point correspondence of the defect in either field; otherwise they are called incongruous (Fig. 7.11).
Unilateral visual inattention refers to the phenomenon found in some patients with parieto-occipital lesions. No field defect is found on unilateral testing, but when stimuli are placed on both right and left hemifields, the patient appears not to see the object on the field opposite to the lesion. Unilateral visual inattention is often seen with incomplete homonymous defects and in the process of recovery of a dense field defect, particularly at the margins of the defect.
Dissociation of the perception of kinetic and static stimuli (Riddoch’s phenomenon) occasionally occurs with occipital lesions, and less often with lesions anywhere in the optic pathways (e.g., optic tract and optic chiasm lesions). In this case, the patient can still appreciate moving objects within a dense field defect for static stimuli [12]. In some cases, nonstriated projections might mediate visual function in the absence of striate cortex (e.g., through superior colliculus-pulvinar-prestriate cortex paths).
Localization of Visual Field Defects
Most important for lesion localization, is to note whether the field defect is monocular, in which case the lesion usually affects the retina or the optic nerve, or binocular, in which case the lesion is localized to or beyond the optic chiasm (Figs. 7.2 and 7.11). Obviously, multiple lesions in the visual pathways, which occur frequently with multiple sclerosis (MS) and other conditions, may result in bilateral loss even when the anterior optic pathways are involved. The pattern of the visual field loss can seldom differentiate retinal from optic nerve disease. However, retinal involvement generally accompanies obvious ophthalmoscopic abnormalities. Also, most optic neuropathies involve visual acuity; spared acuity should raise the suspicion of preretinal, retinal, or retrochiasmal disease [42].
Monocular visual field defects are almost always due to disease of the choroid, retinal pigment epithelium, retina, optic disc, or optic nerve. Lesions affecting the retina, nerve fiber layer, or optic nerve produce visual field defects in the ipsilateral eye, which correspond in position, shape, extent, and intensity to the lesion. Almost all retinal lesions resulting in visual field loss are visible ophthalmoscopically. Careful attention should be directed to the retina and retinal nerve fiber layer corresponding to the visual field defect. Patients with macular disease may also complain of metamorphopsia, micropsia, and positive photopsias (e.g., flashing lights), which are unusual in patients with optic neuropathies.
In assessing optic nerve-related visual field defects, several anatomic points are worth remembering:
1. Fibers from peripheral ganglion cells occupy a more peripheral position of the optic disc, whereas fibers from ganglion cells located closer to the disc occupy a more central position.
2. Peripheral fibers course peripherally through the entire extent of optic nerve.
3. The papillomacular bundle occupies a large sector-shaped region of the temporal disc. This bundle of fibers moves centrally in the more distal (posterior) portions of the orbital optic nerve.
4. All retinal fibers retain their relative positions throughout visual pathways except in the optic tract and at the lateral geniculate nucleus where there is a rotation of 90 degrees that becomes “straightened out” in the optic radiations.
Central visual field defects (unilateral or bilateral) are the result of damage to the papillomacular bundle or optic nerve. Any visual field defect produced by a retinal lesion may be produced by a lesion of the optic nerve [128] and virtually any etiology may be responsible (e.g., glaucomatous, degenerative, ischemic, traumatic, inflammatory, infiltrative, compressive, or vascular optic neuropathy). For example, unilateral central scotomas are often seen with ON, compressive optic neuropathies, or early macular disease. Bilateral central or cecocentral scotomas usually indicate hereditary (e.g., Leber’s hereditary optic neuropathy) or toxic-nutritional optic neuropathies, but may also be seen with bilateral macular lesions, bilateral compressive lesions affecting the optic nerves, or even bilateral lesions affecting the occipital poles. The clinical features and etiologies of bilateral superior and inferior altitudinal defects and bilateral central or cecocentral scotomas are noted in Table 7.1.
TABLE 7.1 Clinical Features and Etiologies of Bilateral Superior or Inferior Altitudinal Defects and Bilateral Central or Cecocentral Scotomas
Although monocular visual field defects are usually due to retinal or optic nerve disease, in the early stages of a chiasmatic lesion, the loss may be restricted to the temporal portion of the field corresponding to the ipsilateral eye [70]. This monocular (often scotomatous) temporal hemianopia (junctional scotoma of Traquair, Fig. 7.12) is attributed to the involvement of the ipsilateral optic nerve close enough to the chiasm to impair conduction selectively in ipsilateral crossing fibers but too anterior to affect nasal retinal fibers crossing from the fellow eye (i.e., nasal compression of the distal intracranial optic nerve ipsilateral to the defect) [70]. Also, lesions located in the most anterior extent of the calcarine cortex cause a crescent-shaped defect restricted to the temporal field of the contralateral eye from 60 to 90 degrees (monocular temporal crescent or “half-moon syndrome”) [26,109]. This is the only retrochiasmatic lesion that may cause a strictly unilateral visual field defect (Fig. 7.11). Similarly, an occipital lesion that spares the foremost part of the calcarine cortex results in a homonymous hemianopia that spares the unpaired temporal crescent (Fig. 7.11).
Monocular altitudinal defects (Fig. 7.2), which are often accompanied by macular sparing, are characteristic of disease in the distribution of the central retinal artery. Central vision may be spared because the blood supply for the macula often derives from the cilioretinal arteries (Fig. 7.1). AION (infarction involving the anterior portion of the optic nerve), due to ischemia involving the posterior ciliary arteries, is another common cause of an altitudinal (usually inferior) defect (see subsequent text). Other causes of a monocular altitudinal defect include choroiditis, choroidal coloboma, retinal detachment, glaucoma, optic nerve hypoplasia, chronic atrophic papilledema, drusen, ON, optic nerve trauma, and masses affecting the optic nerve or chiasm. Bilateral altitudinal defects may result from bilateral lesions, often ischemic, of the retinas or optic nerves, but bilateral occipital lesions, especially trauma or infarction, may also be responsible for this type of defect (Table 7.1) [62,94,106,138]. Rarely, a large prechiasmal lesion compresses both nerves inferiorly to cause bilateral superior altitudinal defects. The compression of nerves from below may also elevate them against the dural shelves extending out from the intracranial end of the optic canals and cause bilateral inferior altitudinal defects. Bilateral lesions of medial aspect of the lateral geniculate body may cause bilateral inferior altitudinal defects. It is important to emphasize that the nerve fiber layer of the retina respects the horizontal meridian only in the nasal field, not in the temporal field; therefore, incomplete altitudinal field defects are more common with retinal lesions. Because of the anastomotic blood supply of the occipital pole, only altitudinal defects due to occipital infarcts spare macular vision [62,106]. Diagnosis of retinal branch artery occlusion or AION is aided by the presence of a unilateral altitudinal defect along with ipsilateral funduscopic changes and, in most bilateral cases, by sequential temporal development; bilateral occipital infarcts are characterized by the sudden, simultaneous onset of altitudinal visual field defects with an absence of retinal or optic nerve abnormality or abnormalities of the pupillary response [106].
FIG. 7.12. Junctional scotoma of Traquair.
Bilateral ring defects may be the consequence of retinal disease, but bilateral occipital involvement can cause a similar field defect. In the case of occipital lesions, however, a vertical step can be regularly identified between the two halves of the ring (Fig. 7.11).
Bitemporal field defects [137] are most often due to a compressive mass lesion affecting the optic chiasm, such as pituitary tumors. Rarely, processes that cause rapidly developing hydrocephalus in children may result in bitemporal defects, perhaps through dilation of the optic recess of the third ventricle. True pure complete bitemporal hemianopias are rare because it is difficult for any pathogenetic mechanism, except trauma, to affect crossing fibers only. Bitemporal hemianopsia may be peripheral, paracentral, or central. The visual field defect may “split” or “spare” the macular central field. Certain anatomical relationships are important in evaluating chiasmal visual field defects:
1. The ratio of crossed to uncrossed fibers in the chiasm is 53:47.
2. Uncrossed fibers, both dorsal and ventral, maintain their relative position at the lateral aspects of the chiasm and pass directly into the ipsilateral optic tract.
3. Dorsal extramacular crossing fibers from each eye decussate posteriorly in the chiasm and then directly enter the dorsomedial aspect of contralateral optic tract.
4. Macular fibers that cross do so in the central and posterior portions of chiasm.
5. Some inferonasal retina fibers, primarily peripheral fibers, loop in Wilbrand’s loop (although the anatomic existence of this structure has been questioned).
Early chiasmal compression with pituitary tumors affecting crossing fibers in isolation usually results in relative rather than absolute defects limited to the central parts of the upper temporal quadrants. With increasing tumor growth, the contact area with the chiasm increases in size so that noncrossing fibers are always affected and acuity is impaired. Therefore, complete bilateral hemianopia almost never occurs in isolation and is usually combined with binasal depression and subnormal acuity [42]. Although most patients with midchiasmal compression demonstrate bitemporal superior visual depression, occasional patients may demonstrate bitemporal scotomas or, rarely, bilateral arcuate defects [48].
Clinically, three chiasmatic syndromes may be recognized (Fig. 7.13) [128].
1. The anterior chiasm or junctional syndrome (different from the junctional syndrome of Traquair, above), in which a unilateral optic defect is associated with a superior temporal defect in the other eye.
2. Body of the chiasm syndrome, in which patients demonstrate bitemporal visual field defects. These visual field defects may be peripheral, central, or a combination of both, with or without “splitting of the macula,” and may be quadrantic or hemianopic. Visual acuity is usually normal, and the optic discs are normal or pale.
3. The posterior chiasm syndrome, in which visual field testing reveals bitemporal scotomas (the peripheral visual fields are intact). Visual acuity and the optic discs are normal.
Lesions affecting the optic chiasm are listed in Tables 7.2 and 7.3. Superior bitemporal field defects may also occur with tilted discs, an optic disc anomaly in which the discs have an elliptical shape. This field anomaly differs from that due to a chiasmatic lesion in that with tilted discs, the defect crosses the median into the nasal field (i.e., does not “respect” the vertical meridian) [47,60]. Pseudochiasmal visual field defects (i.e., bitemporal defects that do not respect the vertical midline) may also be due to ametropia, astigmatism, colobomas, bilateral nasal retinal disease (e.g., schisis), glaucoma, and bilateral optic neuropathies. Rarely a bitemporal hemianopia due to retinal disease can respect the vertical meridian [170].
A central defect in one field with a superior temporal defect in the opposite field points to the involvement of the anterior angle of the chiasm, with damage of the ipsilateral optic nerve and of the loop made by the fibers from the inferonasal retina of the other eye (Wilbrand’s knee) (Figs. 7.7 and 7.13A). Because of its localizing implications, this type of visual field defect has been termed junctional scotoma (different from the junctional syndrome of Traquair, as explained in preceding text) [188]. Such junctional scotomas stress the importance of meticulous testing of the visual fields in the “normal” eye in patients with apparently unilateral visual impairment. As noted in the preceding text, the existence of Wilbrand’s knee has come into question. Nevertheless, whether Wilbrand’s knee exists anatomically, the localizing value of junctional visual field loss to the junction of the optic nerve and chiasm remains undiminished because chiasmal compression alone or ON affecting the junction of the posterior optic nerve and chiasm may result in the contralateral superotemporal visual field defect (junctional scotoma) [71,87].
FIG. 7.13. Visual field defects with chiasm lesions: A: Anterior chiasm or junctional syndrome.B: Body of chiasm syndrome. C: Posterior chiasm syndrome.
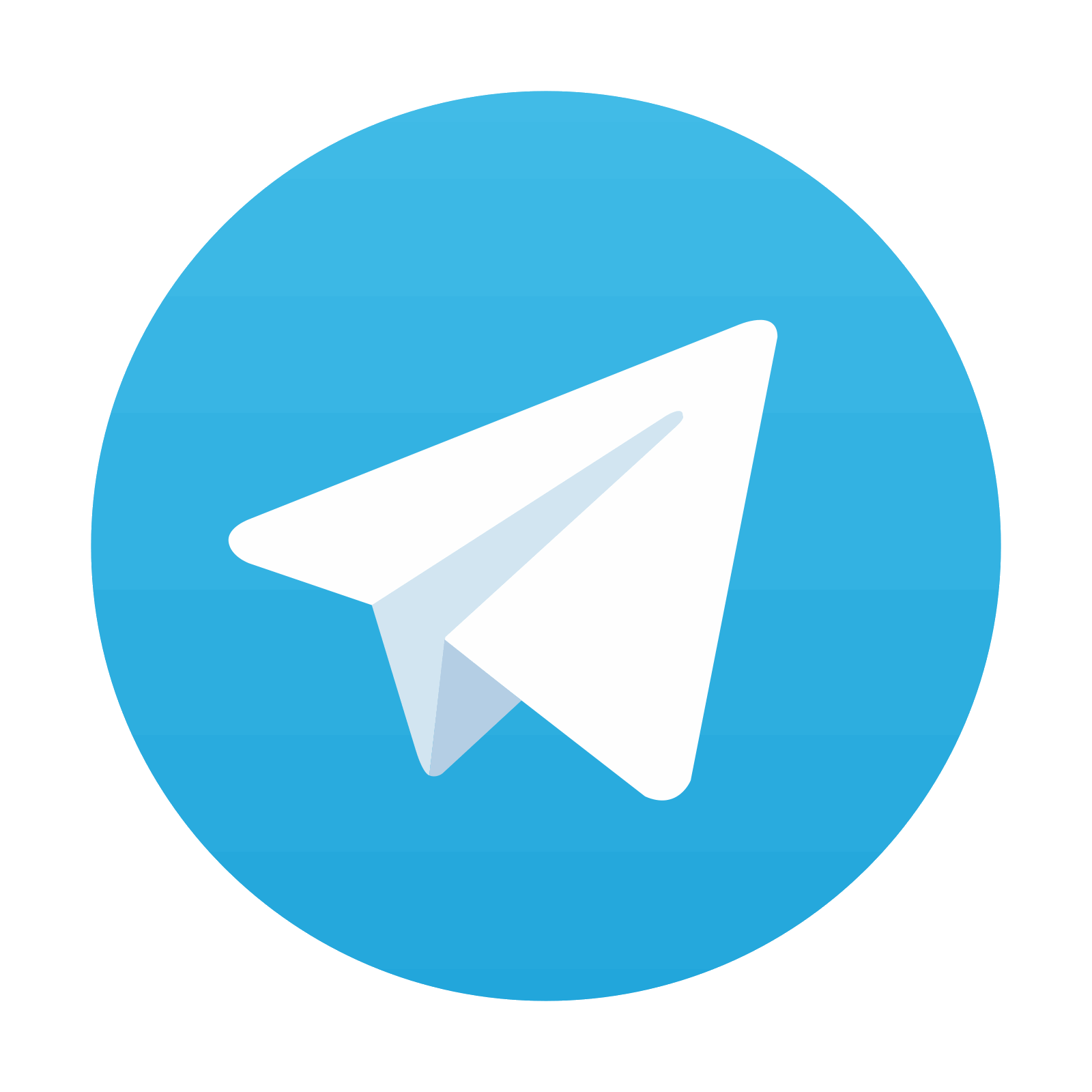