Water Channels
Abstract
Alterations in distinct astrocyte membrane channels, receptors, and transporters have all been associated with the epileptic state. This chapter focuses on the potential roles of the glial water channel aquaporin-4 (AQP4) in modulation of brain excitability and in epilepsy. We will review studies of mice lacking AQP4 (AQP4−/− mice) or α-syntrophin (an AQP4 anchoring protein) and discuss the available human studies demonstrating alterations of AQP4 in human epilepsy tissue specimens. We will conclude with new studies of AQP4 regulation and discuss the potential role(s) of AQP4 in the development of epilepsy (epileptogenesis). While many questions remain unanswered, the available data indicate that AQP4 and its molecular partners may represent important new therapeutic targets.
Keywords
Aquaporin; epilepsy; potassium; seizure; transport; water; aquaporin-4; AQP4
Overview
Recent studies have implicated glial cells in modulation of synaptic transmission, so it is plausible that glial cells may have a functional role in the hyperexcitability characteristic of epilepsy. Indeed, alterations in distinct astrocyte membrane channels, receptors, and transporters have all been associated with the epileptic state. This chapter focuses on the potential roles of the glial water channel aquaporin-4 (AQP4) in modulation of brain excitability and in epilepsy. We will review studies of mice lacking AQP4 (AQP4−/− mice) or α-syntrophin (an AQP4 anchoring protein) and discuss the available human studies demonstrating alterations of AQP4 in human epilepsy tissue specimens. We will conclude with new studies of AQP4 regulation and discuss the potential role(s) of AQP4 in the development of epilepsy (epileptogenesis). While many questions remain unanswered, the available data indicate that AQP4 and its molecular partners may represent important new therapeutic targets.
Water and Ion Homeostasis and Epilepsy
Glial cells are involved in many important physiologic functions, such as sequestration and/or redistribution of K+ during neural activity, neurotransmitter cycling, and provision of energy substrates to neurons [1]. Several recent lines of evidence strongly suggest that changes in glial cells potentially contribute to epilepsy [2–4]. First, many studies now link glial cells to modulation of synaptic transmission [5–9]. Second, functional alterations of specific glial membrane channels and receptors have been discovered in epileptic tissue [10–15]. Third, direct stimulation of astrocytes has been shown to be sufficient for neuronal synchronization in epilepsy models [9] (although see [16]). Thus, if the cellular and molecular mechanisms by which glial cells (especially astrocytes) modulate excitability are better understood, specific antiepileptic therapies based on modulation of glial receptors and channels can be contemplated [17]. It is likely that therapies directed to glial cells would have fewer deleterious side effects than current therapies targeting neurons.
Alteration of water and K+ homeostasis could dramatically affect seizure susceptibility. First, brain tissue excitability is exquisitely sensitive to osmolarity and the size of the extracellular space (ECS) [18]. Decreasing ECS volume with hypoosmolar treatment produces hyperexcitability and enhanced epileptiform activity [19–22]. At least two mechanisms could be operative: decreasing ECS volume could increase extracellular neurotransmitter and ion concentrations and magnify ephaptic interactions among neurons due to increased ECS resistance [3]. Conversely, increasing ECS volume with hyperosmolar treatment powerfully attenuates epileptiform activity [20,22–24]. These experimental data parallel extensive clinical experience indicating that hypoosmolar states such as hyponatremia lower seizure threshold while hyperosmolar states elevate seizure threshold [25–27]. Second, millimolar and even submillimolar increases in extracellular K+ concentration powerfully enhance epileptiform activity in the hippocampus [28–31]. High K+ reliably induces epileptiform activity in hippocampal slices from human patients with intractable temporal lobe epilepsy [32].
Aquaporins
The aquaporins (AQPs) are a family of membrane proteins that function as water channels in many cell types and tissues in which fluid transport is crucial [33–35]. The AQPs are small hydrophobic integral membrane proteins (~30 kDa monomer) that facilitate bidirectional water transport in response to osmotic gradients [36]. Multiple mammalian AQPs have been identified including AQP0, AQP1, AQP2, AQP4, and AQP5 which transport water only (aquaporins), and AQP3, AQP7, and AQP9 which also transport glycerol (aquaglyceroporins) [35,36].
AQP4 [37–39] is of particular interest in neuroscience as it is expressed in brain and spinal cord by glial cells, especially at specialized membrane domains including astroglial endfeet in contact with blood vessels and astrocyte membranes that ensheathe glutamatergic synapses [40–44] (Figs. 8.1 and 8.2). Activity-induced radial water fluxes in neocortex have been demonstrated that may represent water movement via AQP channels in response to physiological activity [45,46]. Interestingly, AQP4 is a structural component of orthogonal arrays of particles (OAPs) seen in freeze-fracture electron micrographs [47–51].

(A) Each AQP4 monomer consists of six membrane-spanning α-helices with both termini located intracellularly. Water selectivity depends on two pore helices and their highly conserved Asn–Pro–Ala motifs. Like other aquaporins, AQP4 forms homotetramers (not shown). (B) Polarization of AQP4 within the astrocytic endfeet at the glial–pial interfaces of the subarachnoid space and the Virchow–Robin space, and the glial–endothelial interface of arteries and arterioles. Astrocytic processes, together with collagen fibers of the subpial space, form the glia limitans externa that seals the brain surface, and form a dense sheath around capillaries, thereby constituting an integral part of the blood–brain barrier. (C) AQP4 is connected to the dystrophin-associated protein complex, which connects the astrocytic cytoskeleton via α1-syntrophin with the basal lamina and is mainly responsible for the polarization of AQP4 at the astrocyte endfeet. AQP4, aquaporin-4; α-DG, α-dystroglycan; β-DG, β-dystroglycan; DP71, dystrophin variant DP71; PDZ, PDZ domain of α1-syntrophin; SXV, Ser-X-Val domain of aquaporin-4; Syn, α1-syntrophin. Source: Reproduced with permission from Jarius S, Paul F, Franciotta D, Waters P, Zipp F, Hohlfeld R, et al. Mechanisms of disease: aquaporin-4 antibodies in neuromyelitis optica. Nat Clin Pract Neurol 2008;4(4):202–14.

In addition to its well-known perivascular location on astrocyte endfeet, AQP4 is also localized in perisynaptic astrocyte processes. Depicted here are the presynaptic (axon) and postsynaptic (dendrite) components of the synapse as well as the perisynaptic astrocyte process (together forming the “tripartite” synapse). Multiple channels and transporters (eg, AQP4, Kir4.1, Na+/K+/ATPase, chloride cotransporters, glutamate transporters) are located on the perisynaptic astrocyte membrane. Source: Reproduced with permission from Binder OK, Nagelhus EA, Ottersen OP. Aquaporin-4 and epilepsy. Glia 2012;60(8):1203–14.
AQP4−/− Mice
AQP4−/− mice were originally generated by targeted gene disruption in 1997 [52], and recently a glial conditional deletion of AQP4 has been generated [53]. AQP4−/− mice are grossly normal phenotypically, do not manifest overt neurological abnormalities, altered blood–brain barrier properties, abnormal baseline intracranial pressure, impaired osmoregulation, or obvious brain dysmorphology [54–56] (Fig. 8.3). A mild hearing impairment has been recorded and the electroretinogram is perturbed [57,58]. Mice with AQP4 deletion have normal blood chemistries and hematologies, normal expression of other brain aquaporins (AQP1 and AQP9) and their only nonneural phenotype is a mild impairment in maximal urine concentrating ability [52].

Nissl stain of coronal section of cortex and dorsal hippocampus reveals no gross histologic abnormalities in AQP4−/− mice. Source: Modified with permission from Binder OK, Oshio K, Ma T, Verkman AS, Manley GT. Increased seizure threshold in mice lacking aquaporin-4 water channels. Neuroreport 2004;15(2):259–62.
Interestingly, more detailed studies of brain tissue from AQP4−/− mice demonstrated reduced osmotic water permeability as measured in isolated membrane vesicles [52], brain slices [59], and intact brain [60]. Also, water permeability was sevenfold reduced in primary astrocyte cultures from AQP4−/− mice as measured by a calcein fluorescence quenching method [61], and similar results were obtained with AQP4 RNAi knockdown experiments in wild-type astrocytes [62]. These data directly demonstrated that AQP4 provides the predominant pathway for transmembrane water movement in astrocytes.
In vivo studies of these mice demonstrated a functional role for AQP4 in brain water transport. AQP4−/− mice have markedly decreased accumulation of brain water (cerebral edema) following water intoxication and focal cerebral ischemia [63] and impaired clearance of brain water in models of vasogenic edema [56]. Clearance of seizure-induced edema may also be AQP4-dependent [64]. Impaired water flux into (in the case of cytotoxic edema) and out of (in the case of vasogenic edema) the brain makes sense based on the bidirectional nature of water flux across the AQP4 membrane channel at the blood–brain barrier. The recently generated glial-conditional AQP4 knockout mouse line demonstrates a 31% reduction in brain water uptake after systemic hypoosmotic stress [53]. Similarly, mice deficient in dystrophin or α-syntrophin, in which there is mislocalization of the AQP4 protein [65–67], show attenuated cerebral edema [66,68].
Altered ECS in AQP4−/− Mice
The ECS in brain comprises ~20% of brain tissue volume, consisting of a jelly-like matrix in which neurons, glia, and blood vessels are embedded [69]. The ECS contains ions, neurotransmitters, metabolites, peptides, and extracellular matrix molecules, forming the microenvironment for all cells in the brain and mediating glia–neuron communication via diffusible messengers, metabolites, and ions [70]. The size of the ECS is likely largely controlled by astrocytic mechanisms [71–73].
The ECS of AQP4−/− mice was assessed using a cortical fluorescence recovery after photobleaching (cFRAP) method to measure the diffusion of fluorescently-labeled macromolecules in the cortex [74]. ECS in mouse brain was labeled by exposure of the intact dura to fluorescein dextrans (Mr 4, 70, and 500 kD) after craniectomy, and fluorescein-dextran diffusion was detected by fluorescence recovery after laser-induced cortical photobleaching using confocal optics. The cFRAP method was applied to brain edema, seizure initiation, and AQP4 deficiency. In contrast to the slowed diffusion produced by brain edema and seizure activity, ECS diffusion was faster in AQP4−/− mice, indicating ECS expansion in AQP4 deficiency [74]. Similar results were obtained with follow-up studies using the TMA+ method [75].
Seizure Phenotype of AQP4−/− Mice
Seizure susceptibility of AQP4−/− mice was initially examined using the convulsant (GABAA antagonist) pentylenetetrazol (PTZ) [55]. At 40 mg/kg PTZ (i.p.), all wild-type mice exhibited seizure activity, whereas six out of seven AQP4−/− mice did not exhibit seizure activity. At 50 mg/kg PTZ, both groups exhibited seizure activity; however, the latency to generalized (tonic-clonic) seizures was longer in AQP4−/− mice [55]. Since seizure propensity is exquisitely sensitive to ECS volume [18], the expanded ECS in AQP4 deficiency is consistent with the increased seizure threshold. Thus, more intense stimuli (eg, higher PTZ doses or a longer time after PTZ) may be required to overcome the expanded ECS of AQP4−/− mice in order to initiate a seizure.
In order to analyze the seizure phenotype of the AQP4−/− mice in greater detail, in vivo electroencephalographic (EEG) characterization with stimulation and recording was employed [76] (Fig. 8.4). AQP4−/− mice and wild-type controls were implanted in the right dorsal hippocampus with bipolar electrodes. Following postoperative recovery, electrical stimulations were given to assess electrographic seizure threshold and duration. AQP4−/− mice had a higher mean electrographic seizure threshold than wild-type controls, consistent with the prior PTZ studies [55]. However, AQP4−/− mice were also found to have remarkably prolonged stimulation-evoked seizures compared to wild-type controls [55].

(A) Bipolar electrodes implanted in the right hippocampus were connected to a stimulator and digital EEG acquisition system. Mice were awake and behaving normally at the onset of stimulation (inset). (B) Representative electroencephalograms from WT and AQP4−/− mice. Baseline EEG prior to stimulation is similar (left). Hippocampal stimulation-induced electrographic seizures are shown for a WT mouse (top) and an AQP4−/− mouse (bottom). The WT mouse had a 11-second seizure, whereas the AQP4−/− mouse had a much longer seizure (37 seconds). Behavioral arrest was observed in both animals during the seizure. Postictal depression is evident on the EEG in both cases. (C) Electrographic seizure threshold (μA) (mean ±SEM) in WT versus AQP4−/− mice. AQP4−/− mice had a higher electrographic seizure threshold than wild-type controls. (D) Electrographic seizure duration (seconds) (mean ±SEM) following hippocampal stimulation in WT versus AQP4−/− mice. AQP4−/− mice had remarkably longer stimulation-evoked seizures compared to wild-type controls. **, P < 0.01; ***, P < 0.001 compared to WT. Source: Reproduced with permission from Binder DK, Yao X, Sick TJ, Verkman AS, Manley GT. Increased seizure duration and slowed potassium kinetics in mice lacking aquaporin-4 water channels. Glia 2006;53:631–6.
Altered K+ Homeostasis in AQP4−/− Mice
Because of the colocalization of AQP4 and inwardly-rectifying K+ channels in glial endfeet, the hypothesis arose that AQP4 was indirectly involved in K+ reuptake [40,77]. Impaired K+ clearance from the ECS following the intense neuronal activity accompanying the seizure would lead to prolonged depolarization of neurons and inhibit seizure termination [10,28–30]. Indeed, in addition to modulation of brain water transport, AQP4 and its known molecular partners have been hypothesized to modulate ion homeostasis [78,79]. During rapid neuronal firing, extracellular [K+] increases from ~3 mM to a maximum of 10–12 mM; and K+ released by active neurons is thought to be primarily taken up by glial cells [69,80–82]. Such K+ reuptake into glial cells could be AQP4-dependent, as water influx coupled to K+ influx is thought to underlie activity-induced glial cell swelling [83,84]. In support of this possibility was the known subcellular colocalization of AQP4 with the inwardly-rectifying K+ channel Kir4.1 in the retina [41,77,85]. Kir4.1−/− mice, like AQP4−/− mice [57,58], demonstrate abnormal retinal and cochlear physiology presumably due to altered K+ homeostasis [86–89]. Kir4.1 is thought to contribute to K+ reuptake and spatial K+ buffering by glial cells [90–93], and pharmacological or genetic inactivation of Kir4.1 leads to impairment of extracellular K+ regulation [86,94–99].
To address the possibility that AQP4 deficiency was associated with a deficit in K+ homeostasis, K+ dynamics were examined in vivo in AQP4−/− mice [76] (Fig. 8.5). Neither baseline [K+]o nor the “Lux-Heinemann ceiling” level of activity-induced [K+]o elevation (~12 mM) [80,81] were altered in AQP4 deficiency, indicating that basic K+ homeostasis was intact. Based on K+ measurements made from cortex with double-barreled K+-sensitive microelectrodes, stimulation-induced rises in [K+]o were quite different in AQP4−/− compared to wild-type mice; in particular, there was a markedly slower rise and decay time for poststimulus changes in [K+]o in AQP4−/− mice [76] (Fig. 8.5). A similar delay in K+ kinetics was observed following cortical spreading depression in AQP4−/− mice using a fluorescent K+ sensor [100].

(A) Double-barreled potassium-selective microelectrodes were introduced through a small craniectomy and connected to a high-impedance electrometer for real-time [K+] acquisition. A bipolar electrode connected to a digital stimulator was positioned so as to provide direct current stimulation of the cortex at varying intensities. (B) Sample calibration curve for a K+-sensitive microelectrode placed in serial K+ standard solutions. (C) Representative family of K+ curves in WT mouse following stimulation intensities of 1, 5, 10, and 20 μA. (D) Representative 20 μA stimulation curves from three different WT mice (top) and three different AQP4−/− mice (bottom). Calibration in (C) also applies to (D). Source: Reproduced with permission from Binder DK, Yao X, Sick TJ, Verkman AS, Manley GT. Increased seizure duration and slowed potassium kinetics in mice lacking aquaporin-4 water channels. Glia 2006;53:631–6.
Slowed [K+]o rise time is consistent with increased ECS volume fraction in AQP4−/− mice [74]. Slowed [K+]o decay is possibly due to impaired K+ reuptake into AQP4−/− astrocytes. Interestingly, there is no difference in expression of Kir4.1 protein [76] or Kir4.1 immunoreactivity [101] in AQP4−/− mice nor AQP4 immunoreactivity in Kir4.1−/− mice [101]. In addition, no alterations were observed in membrane potential, barium-sensitive Kir4.1 K+ current or current–voltage curves in AQP4−/− retinal Müller cells [102] or brain astrocytes [103]. Lack of alteration of Kir channels in AQP4−/− mice suggests the interesting possibility that the slowed [K+]o decay may be a secondary effect of slowed water extrusion (“deswelling”) following stimulation, and this has been modeled carefully [104] but not directly demonstrated.
Stroschein et al. [105] investigated the impact of AQP4 on stimulus-induced alterations of [K+]o in hippocampal slices. Antidromic stimulation evoked smaller increases and slower recovery of [K+]o in the stratum pyramidale of AQP4−/− mice, consistent with the previous in vivo studies in cortex. Interestingly, astrocyte gap junction coupling as assessed with tracer filling during patch-clamp recording demonstrated enhanced tracer coupling in AQP4−/− mice, and laminar profiles indicated enhanced spatial redistribution of K+ [105]. The functional consequences of alterations in gap junctional coupling are not completely clear; however, the complete absence of astrocytic gap junctions impairs K+ homeostasis [106]. To further assess the direct link between AQP4 and K+ homeostasis, Haj-Yasein et al. examined extracellular K+ concentration in a distinct constitutive AQP4−/− mouse line [107] and found a layer-specific effect of AQP4 deletion on K+ dynamics during synaptic stimulation [108].
Anchoring of AQP4 by the Dystrophin-Associated Protein Complex
AQP4 in endfeet membranes is anchored to the dystrophin-associated protein complex (DAPC) (Fig. 8.1C). For example, endfoot expression of AQP4 is dependent on α-syntrophin, a dystrophin-associated protein [67]. Accordingly, mice deficient in α-syntrophin show marked loss of AQP4 from perivascular and subpial membranes as judged by quantitative immunogold electron microscopy [68]. Similarly, dystrophin-deficient (mdx) mice exhibit a dramatic reduction of AQP4 in astroglial endfeet surrounding capillaries and at the glia limitans (cerebrospinal fluid–brain interface) despite no alteration in total AQP4 protein [66]. These studies clearly suggest that alterations in components of the DAPC may affect the subcellular targeting and function of AQP4. A recent study also demonstrates that dystrophin localization at the astrocytic endfoot is dependent on syntrophin [109]. Another study using the zero magnesium in vitro model of epilepsy demonstrated reduction in expression of a distinct member of the DAPC, β-dystroglycan, in cortical astrocytes following continuous seizures, which was accompanied by a decrease in AQP4 expression [110]. Astrocyte swelling is markedly impaired in response to hypoosmotic stress, oxygen-glucose deprivation or high K+ in the cortex of α-syntrophin-negative GFAP/EGFP mice [111].
What are the functional consequences to seizure susceptibility of loss of DAPC components? Amiry-Moghaddam et al. [4] were the first to report a possible association between AQP4 and epilepsy by demonstrating an increased severity of hyperthermia-evoked seizures in α-syntrophin-deficient mice. These mice exhibited a deficit in extracellular K+ clearance following evoked neuronal activity. Dystrophin-deficient mice were found to have altered seizure susceptibility in response to various chemical convulsants; in particular, mdx mice showed enhanced seizure severity and a shorter latency in the development of chemical kindling produced by administration of PTZ [112]. It is interesting to note that there is an increased incidence of epilepsy in forms of human muscular dystrophy in which the dystrophin complex is affected [113]. Taken together, these data suggest that AQP4 and its molecular partners together comprise a multifunctional “unit” responsible for clearance of K+ and/or water following neural activity and that alterations in expression of this complex can lead to alterations in seizure susceptibility.
Human Tissue Studies
The most common pathology in patients with medically-intractable temporal lobe epilepsy is mesial temporal sclerosis (MTS), characterized by marked neuronal cell loss in specific hippocampal areas, gliosis, and microvascular proliferation [114]. Emerging work also demonstrates dysregulation of water and K+ homeostasis in patients with mesial temporal lobe epilepsy. First, imaging studies demonstrate abnormal T2 prolongation by magnetic resonance imaging (MRI) in the epileptic hippocampus, possibly partially due to increased water content [115]. This is accompanied by alterations in apparent diffusion coefficient (ADC) with diffusion-weighted MRI imaging [116]. Second, the expression and subcellular localization of AQP4 have been shown to be altered in sclerotic hippocampi obtained from patients with MTS. Using immunohistochemistry, rt-PCR and gene chip analysis, Lee et al. demonstrated an overall increase in AQP4 expression in sclerotic epilepsy tissue [117]. However, using quantitative immunogold electron microscopy, the same group found that there was mislocalization of AQP4 in the human epileptic hippocampus, with reduction in perivascular membrane expression [118] (Fig. 8.6). Thus, although there was an overall increase in AQP4 content by Western blot, rt-PCR, and gene chip analysis, the subcellular distribution of AQP4 in mesial temporal lobe epilepsy tissue had changed. Their hypothesis was that reduction in perivascular AQP4 expression would lead to water and K+ dysregulation in the epileptic hippocampus, potentially contributing to hyperexcitability.

Although AQP4 is preferentially distributed around blood vessels in the non-MTLE hippocampus, this localization is lost in MTLE. AQP4 is demonstrated by preembedding immunohistochemistry on Vibratome sections of a representative non-MTLE (A and D) and MTLE (B and C) hippocampus. (A) In the non-MTLE hippocampus, immunoreactivity for AQP4 in the pyramidal layer of Ammon’s horn (the area within the dashed line) is preferentially distributed around blood capillaries (scale bar, 1 mm). (D) This finding is demonstrated in the high-power field of CA1, where the arrow indicates a strongly immunopositive capillary amidst a weakly labeled neuropil (magnification, ×6 selected portion of A). (B) In the MTLE hippocampus, the preferential distribution of AQP4 around blood capillaries is lost in the pyramidal layer in areas of sclerosis (such as CA1). Scale is the same as in (A). (C) In the high-power field of CA1, moderate immunolabeling for AQP4 is present throughout the neuropil and also around blood capillaries, which are indicated by arrows (magnification, ×6 selected portion of B). Source: Reproduced with permission from Eid T, Lee TS, Thomas MJ, Amiry-Moghaddam M, Bjornsen LP, Spencer DD, et al. Loss of perivascular aquaporin-4 may underlie deficient water and K+ homeostasis in the human epileptogenic hippocampus. Proc Natl Acad Sci USA 2005;102(4):1193–8.
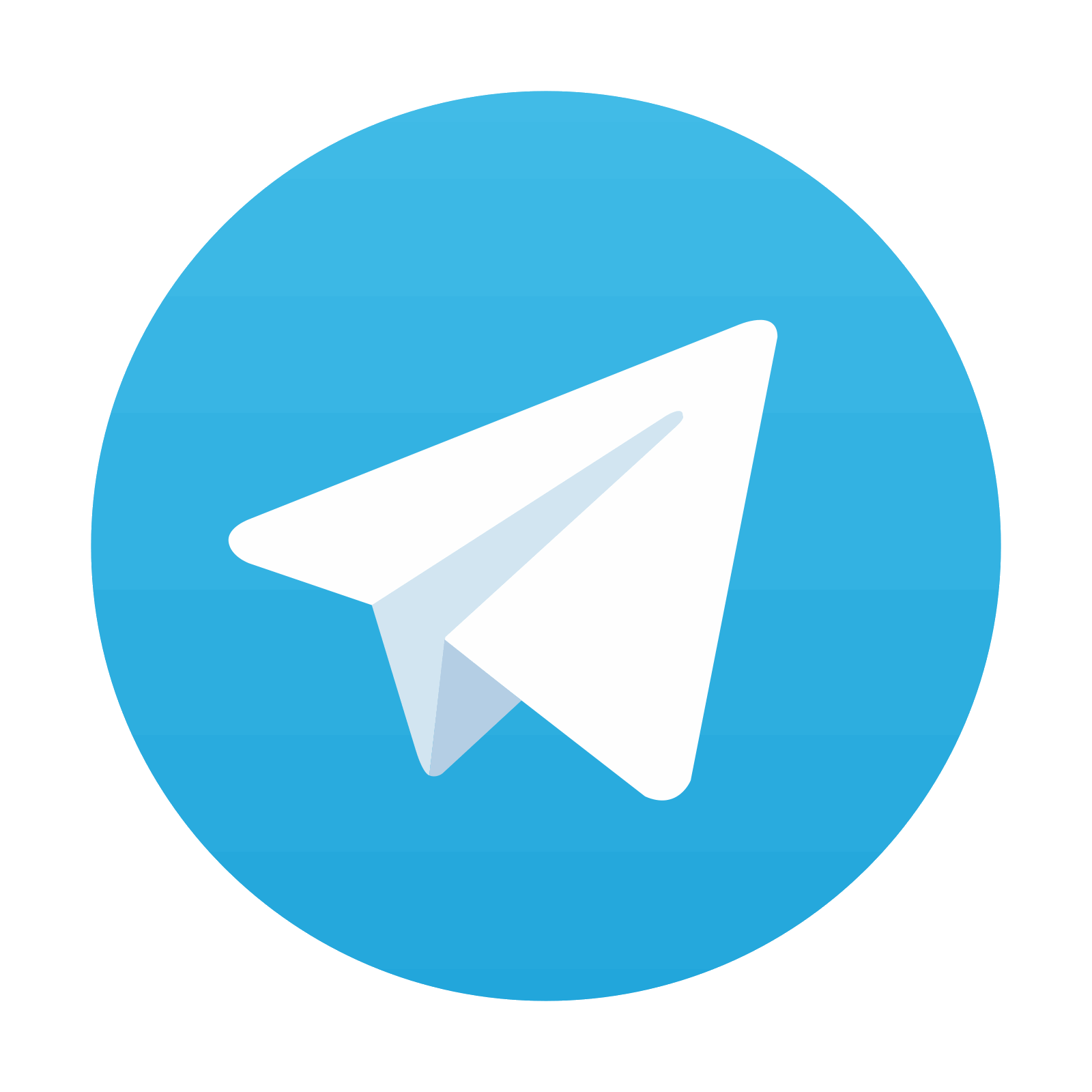
Stay updated, free articles. Join our Telegram channel
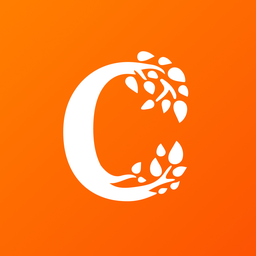
Full access? Get Clinical Tree
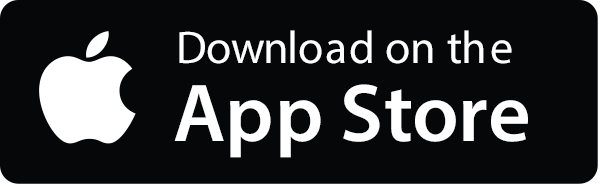
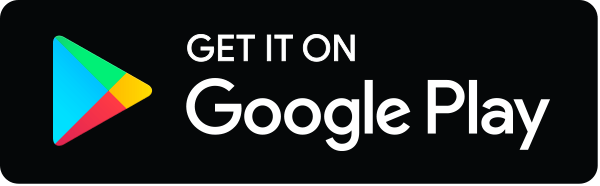
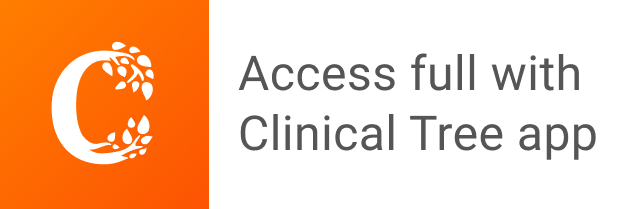