Chapter 80 Bacterial Infections of the Nervous System
The brain is normally a sterile site that is protected from infection by specialized barriers, including the bony skull and the blood–brain barrier. Consequently, infections of the central nervous system (CNS) are comparatively rare. In the United States, the incidence of bacterial meningitis, the most frequent bacterial infection of the CNS, was 3–5 cases per 100,000 population in 1995 [Schuchat et al., 1997], in stark contrast to the incidence of sepsis, which was more than 200 cases per 100,000 in the same year [Martin et al., 2003].
Acute Bacterial Meningitis
Bacterial meningitis is an inflammation of the leptomeninges, triggered by bacteria present in the subarachnoid space. Children still die or suffer permanent neurologic sequelae as a result of meningitis [De Louvois et al., 2007]. Prevention or prompt diagnosis and aggressive management are the goals [Schaad, 1997]. Before the discovery of antibiotics, therapy consisted of withdrawal of cerebrospinal fluid (CSF) through repeated lumbar puncture and intrathecal or intravenous administration of antimeningococcal antiserum raised in horses. More recent milestones include the introduction of new, potent, bactericidal antibiotics, active vaccination, and anti-inflammatory therapy [Tunkel et al., 2004].
Epidemiology
Bacterial meningitis has a unique epidemiologic profile. The disease has a worldwide distribution, the typical host is a previously healthy patient, the typical symptoms usually develop rapidly, there are numerous and severe sequelae, and the disease is increasingly preventable [Schuchat et al., 1997]. Bacterial meningitis occurs in people of all ages, but there are striking age-related differences in the predominant etiologic agents. Differences relate to immunologic maturation, social or behavioral influences, and the coexistence of other chronic or acute clinical conditions.
Neonatal meningitis typically follows obstetric complications, and the predominant bacteria are group B streptococcus, Listeria monocytogenes, and Gram-negative enteric bacteria. Older infants have substantial risk of meningitis caused by Haemophilus influenzae type b and Streptococcus pneumoniae, although where H. influenzae conjugate vaccines are in use, H. influenzae type b meningitis has been virtually eliminated. School-age children are at greatest risk of meningococcal disease, which occurs in outbreaks throughout the world and causes major epidemics in sub-Saharan Africa and parts of Asia. S. pneumoniae is the leading cause of bacterial meningitis in adults, and the emergence of antimicrobial-resistant pneumococci has complicated pediatric meningitis worldwide [Schuchat et al., 1997].
Bacterial meningitis occurs with increased frequency in certain population groups. Native Americans and aboriginal populations are at particular risk for H. influenzae type b disease [Coulehan et al., 1984; Ward et al., 1986]. Risk of group B streptococcal disease is substantially higher among African Americans than other racial or ethnic groups in the United States [Zangwill et al., 1992].
Institutional settings, such as daycare centers, provide opportunities for enhanced transmission of respiratory pathogens, including H. influenzae type b, meningococcus, and pneumococcus. Transmission of antimicrobial-resistant infections among daycare center attendees is an emerging problem [Reichler et al., 1992]. Meningococcal meningitis clusters have been described in schools, on college campuses, and in refugee settings.
Invasive pneumococcal disease, including pneumococcal meningitis, has declined among children and adults since the introduction of the pediatric heptavalent pneumococcal conjugate vaccine (PCV7) (USA in 2000, Europe in 2001–2004) [Tsai et al., 2008]. Although the overall effect of the vaccine remains substantial, a recent increase in meningitis caused by non-PCV7 serotypes, including strains nonsusceptible to antibiotics, is a concern [Hsu et al., 2009]. Pneumococcal serotype 19A has emerged as most frequent cause of invasive pneumococcal disease in children in various regions [Pelton et al., 2007]. In children with pneumococcal meningitis, infections with non-PCV7 serogroups seem less likely to result in death. Among survivors, there is preliminary evidence of parity in neurologic sequelae between PCV7 and non-PCV7 serogroups [Rajasingham et al., 2008].
Pathogenesis
From the nasopharyngeal surface, encapsulated organisms cross the epithelial cell layer and invade the small subepithelial blood vessels. In the bloodstream, pathogens are protected from complement-mediated bactericidal mechanisms and neutrophil phagocytosis by their capsule. Impairment of the alternative complement pathway, sickle cell disease, and lack of a functional spleen predispose to the development of pneumococcal meningitis. Similarly, functional deficiencies of components involved in activation and function of complement-mediated defenses (i.e., mannose binding lectin, properdin, lack of terminal complement components) increase the susceptibility for invasive meningococcal infections [Van Deuren et al., 2000]. Neonates are at an increased risk of bacterial meningitis because of impaired nonspecific immunity. This impaired immunity includes defects in leukocyte chemotaxis, phagocytosis, bactericidal activity, and low levels of immunoglobulin M (IgM) antibodies and complement, which are not transferred across the placenta but are important in defense against Gram-negative organisms [Mariscalco et al., 2002].
Studies in models of bacterial meningitis indicate that cells of the choroid plexus and cerebral capillaries possess receptors for meningeal pathogens. The interaction between these host cell receptors (e.g., 37kd laminin receptor protein, platelet-activating factor receptor) and bacterial ligands, such as outer membrane proteins or pili, are necessary for the bacterial invasion of the CSF space [Orihuela et al., 2009; Kim, 2010]. The interaction between host-cell receptor and bacterial ligand triggers intracellular pathways, upon which pathogens can traverse the blood–brain barrier either transcellularly or paracellularly. Some pathogens, such as L. monocytogenes, may also be transported into the CSF by macrophages [Kim et al., 2005; Kim, 2010]. Not all bacterial infections of the CNS are the result of bacteremia. Nonhematogenous invasion of the CSF by bacteria occurs in situations of compromised integrity of the barriers surrounding the brain (e.g., in otitis media, mastoiditis, sinusitis). Direct communication between the subarachnoid space and the skin or mucosal surfaces as a result of malformation or trauma gives rise to meningeal infection. Bacteria also can reach the CSF as a complication of neurosurgery, spinal anesthesia, or ventriculostomy placement [Alleyne et al., 2000]. Infections of CSF shunts are another cause of meningitis. Most cases occur shortly after implantation of the shunt and are attributed to intraoperative inoculation, but late shunt infections can occur several years after implantation [Vinchon et al., 2002]. Staphylococci, particularly coagulase-negative staphylococci, are the major pathogens in shunt infections [Filka et al., 1999]. These organisms have unique capabilities to colonize the surface of foreign bodies because of specialized surface proteins (e.g., clumping factor, fibronectin binding protein) that allow the organism to adhere to the catheters – in particular, after they are coated with host-derived proteins, such as fibrin and fibronectin [Ziebuhr et al., 2000]. Adhering organisms rapidly develop into a biofilm, characterized by bacteria embedded in an extracellular matrix. Elimination of bacterial biofilms from the surface of foreign bodies by antibiotics or host defenses is notoriously difficult [Ziebuhr et al., 2000]. Colonization of CSF shunts with coagulase-negative staphylococci leads to clinically apparent meningitis or ventriculitis in only approximately 30 percent [Odio et al., 1984; Vinchon et al., 2002].
Pathogens reaching the CSF are likely to survive because of a paucity of resident macrophages and deficient opsonization caused by low concentrations of capsule-specific immunoglobulins and complement in the CSF. Lack of opsonization greatly reduces the effectiveness of incoming granulocytes and allows largely unrestricted multiplication of the meningeal pathogens. Bacterial multiplication is associated with the release of bacterial products (fragments of cell wall, lipopolysaccharide) that trigger the inflammatory response in the subarachnoid space by inducing the production and release of inflammatory cytokines and chemokines. Cytokines, including tumor necrosis factor-α, interleukin-1, and interleukin-6; chemokines, such as interleukin-8, growth-related protein-α, and monocyte chemotactic protein-1; and lipid inflammatory mediators, such as platelet activation factor, upregulate adhesion molecules on brain vascular endothelial cells and promote the recruitment of granulocytes into the CSF. Matrix metalloproteases contribute to the inflammatory process by mediating breakdown of the blood–brain barrier and activating cytokines [Kieseier et al., 1999]. This granulocytic inflammation is primarily responsible for the complex pathophysiologic CNS alterations and clinical manifestations of bacterial meningitis [Meli et al., 2002].
Diagnosis
Prompt diagnosis is crucial for a life-threatening and treatable illness such as bacterial meningitis. Routine early diagnosis requires educated suspicion of meningitis and immediate performance of lumbar puncture and adequate tests when the CSF has been obtained [Schaad, 1995].
Clinical Features
The sudden onset of a stiff neck with fever in a child who appears ill usually indicates the presence of meningitis (Box 80-1). In these cases, a lumbar puncture is a crucial part of the initial evaluation.
Meningismus
Meningismus reflects tense neck and back muscles as a reflex to avoid painful extension of inflamed meninges. Clinical findings of meningeal irritation include Kernig’s sign (passive extension of the knee from the flexed thigh position elicits pain in the back), Brudzinski’s sign (passive flexion of the neck elicits spontaneous flexion of the lower extremities), tripod sign (while sitting, the patient holds the back erect by placing both extended arms behind the buttock), and knee-kissing sign (the patient is unable to kiss his or her own knees). Nuchal rigidity is absent throughout the course of meningitis in only 1–2 percent of infants and children; generally, the younger the patient, the less prominent nuchal rigidity [Geiseler and Nelson, 1982].
Initial presentation
Fever (94 percent), vomiting (82 percent), and nuchal rigidity (77 percent) are the most common presenting symptoms of meningitis in children 1–4 years old. Many children also experience lethargy, irritability, anorexia, and photophobia [Ashwal et al., 1993]. Seizures, bulging fontanel, and coma occur less commonly and usually are seen later in the course of the illness. Older patients report headache and neck pain.
Two modes of presentation
The first pattern develops over several days, with fever and nonspecific manifestations suggesting viral infection without or with localization (e.g., upper respiratory or gastrointestinal tract). In these patients, it is difficult to pinpoint the exact onset of meningitis. The second pattern is acute, sometimes fulminant, and the symptoms and signs of sepsis (e.g., cardiovascular instability; cutaneous manifestations, such as an erythematous, maculopapular, or petechial rash) and meningitis evolve rapidly over a few hours [Thompson et al., 2006].
Neurologic findings
Seizures occur before hospital admission or within the first 2 days of hospitalization in 20–30 percent of pediatric patients with bacterial meningitis [Feigin et al., 1992]. Generalized seizures usually are not a predictor of poor outcome. In contrast, focal neurologic signs, such as paresis, cranial nerve palsy, and focal seizure activity, often precede more adverse outcomes of irreversible brain damage. Severely depressed consciousness at the time of hospital admission usually signals a poor prognosis because of the likelihood of extensive encephalitic involvement or massively increased intracranial pressure in these patients [Tunkel and Scheld, 1995].
Arthritis and other systemic manifestations
Arthritis can precede or follow H. influenzae and Neisseria meningitidis meningitis, and confuses the neurologic examination by producing the superficial appearance of a monoparesis. Knees and elbows are common sites for arthritis. Of patients with H. influenzae type b septic arthritis, approximately 30 percent have concurrent meningitis [Rotbart and Glode, 1985]. Periorbital cellulitis occurs in H. influenzae meningitis.
Differential diagnosis
The differential diagnosis of bacterial meningitis includes aseptic meningitis, encephalitis, brain abscess, febrile seizure, head trauma, intracranial hemorrhage, and neoplastic meningitis. In addition, unusual infectious agents, such as fungus, rickettsia, or tuberculosis, must be considered. A fever with a simple seizure is unlikely to be bacterial meningitis [Green et al., 1993].
Neonatal meningitis
The traditional clinical signs of meningitis are usually lacking in neonates. Nuchal rigidity rarely occurs. Fever and a full fontanel may be absent. An associated sepsis is common. Bacterial meningitis should be considered at any age (so-called early and late onset) in any newborn who fails to thrive and has irritability, apnea, seizures, a tendency to opisthotonos, poor feeding, emesis, hypothermia or hyperthermia, hypotonia or hypertonia, a gray appearance, jaundice, or other evidence of sepsis. Apnea may be the only sign of meningitis in a neonate [Heath et al., 2003; Schaad, 1992].
Shunt infections
Infections of CNS shunt occur in approximately 5–10 percent of patients, usually within 1–2 months of surgical insertion [McGirt et al., 2003; Ronan et al., 1995]. Most of these infections are due to coagulase-negative Staphylococcus or Staphylococcus aureus; however, a variety of other organisms, including Gram-negative bacteria and other skin flora, can be causative.
Diagnosis of CNS shunt infection versus CNS shunt malfunction often represents a dilemma in children who present with constitutional signs and symptoms [McClinton et al., 2001]. Clinical manifestations of CNS shunt infection usually include low-grade fever, vomiting, irritability or lethargy, and other signs of increased intracranial pressure. The onset is often insidious but can be more acute, particularly if shunt malfunction also is present. Neck stiffness is uncommon. Shunt infection should be suspected in any child with an indwelling CNS drainage system and fever.
Meningitis after cochlear implants
Cochlear implant is the standard treatment for patients with severe to profound hearing loss, in which well-fit hearing aids fail to permit effective oral communication. The implant is a neural stimulator whose electrode array is placed surgically in the lumen of the cochlea, near the auditory nerve. An external microphone picks up speech signals, and the signal processor transforms these signals into digital impulses that a radiofrequency carrier transmits percutaneously into the internal receiver-stimulator and electrode array. The auditory cortex is stimulated by the implant, permitting the perception of the digitally processed information as speech [Gates and Miyamoto, 2003].
Complications from cochlear implantation are unusual. Reports of postoperative meningitis have called attention to a previously unrecognized risk [Arnold et al., 2002; Reefhuis et al., 2003]. Most cases of meningitis occur in children with a typical presentation. Available information indicates that the mode for infection is a time-limited or, rarely, persistent CSF leak into the middle ear. Bacteria from the middle ear – usually causing otitis media – may enter the meninges. The most common offending pathogens are S. pneumoniae and H. influenzae.
Lumbar Puncture
The diagnosis of bacterial meningitis is based on examination of CSF [Feigin et al., 1992]. Minimizing sequelae of bacterial meningitis depends on the prompt initiation of effective antibiotic therapy. A lumbar puncture should therefore be performed whenever there is even slight clinical evidence of meningitis. Rarely, other sites may be used to obtain CSF, including ventricles or shunts installed to divert CSF.
Lumbar puncture is a safe procedure for most patients. Minor discomfort may occur, but serious consequences are rare. Headache 1–6 hours after lumbar puncture is a troublesome but benign complication. When present, the headache is frontal and is exaggerated by movement from a lying to a sitting or standing position. Headache after lumbar puncture seems to be caused by continued leak of CSF from the site of the lumbar puncture, with resulting low CSF pressure and traction on pain-sensitive structures. The problem can be mitigated by removal of a minimal amount of fluid and use of a small-bore needle [Lynch et al., 1992; Lavi et al., 2010]. Pain in the low back or legs occurs occasionally after lumbar puncture; this pain is of uncertain etiology and resolves spontaneously in several days.
Herniation of the brainstem and cerebellar tonsils into the foramen magnum is extremely rare in children [Marton and Gean, 1986]. A risk of herniation is clearly related to substantially and focally increased intracranial pressure [Richards and Towu-Aghantse, 1986]. The risk of producing meningitis by performing a lumbar puncture in a child with septicemia is insignificant and is not a contraindication to the test [Shapiro et al., 1986].
With the widespread introduction of highly effective bacterial conjugate vaccines against H. influenzae type b and S. pneumoniae, there has been a significant decrease in the incidence of bacterial meningitis in children. This has reduced the probability that a child with CSF pleocytosis will have bacterial meningitis. Several studies were able to validate a previously developed clinical prediction rule, the so-called Bacterial Meningitis Score, for identifying children with CSF pleocytosis at very low risk of bacterial meningitis [Nigrovic et al., 2007]. Patients are classified as very low risk if they lack all of the following criteria: positive CSF Gram stain, CSF absolute neutrophil count of at least 1000 cells/μL, CSF protein of at least 80 mg/dL, peripheral blood absolute neutrophil count of at least 10,000 cells/μL, and a history of seizure before or at the time of presentation.
Laboratory tests
The initial diagnosis of meningitis is based on examination of CSF. Bacterial infection is suggested by increased opening and closing pressure; a cloudy (i.e., not clear) CSF; or a CSF pleocytosis with polymorphonuclear predominance, increased protein and lactate concentration, or decreased glucose concentration. Details and normal values have been described previously (Table 80-1) [Ahmed et al., 1996; Kim, 2010].
Confirmation of the diagnosis requires the detection in the CSF of a specific bacterial pathogen by microscopy or a positive culture, polymerase chain reaction, or rapid antigen-detection test of CSF. Smears of CSF should be examined by Gram stain (acid-fast stain if tuberculosis is suspected). Gram-stained smears of the CSF sediment reveal bacteria in 70–90 percent of untreated cases of meningitis caused by S. pneumoniae or N. meningitidis, but only in one-third of cases caused by L. monocytogenes [Kim, 2010]. The CSF should be cultured on a blood agar plate, on a chocolate agar plate (or on Fildes or Leventhal medium), and in broth. Special cultures for mycobacteria, fungi, or other microorganisms should be undertaken if clinically indicated. In an area of increasing antimicrobial resistance, adequate in vitro susceptibility testing must be routine for any cultured neonatal pathogen. Various antigen-detection tests are available (e.g., countercurrent immunoelectrophoresis, latex particle agglutination, enzyme-linked immunosorbent assay) and may be helpful in establishing an etiologic diagnosis rapidly.
Polymerase chain reaction and other sequence-based microbial detection methods are being used increasingly in many clinical microbiology laboratories. These tests improve detection of pathogens, particularly in patients pretreated with antibiotics [Fredricks and Relman, 1999; Chiba et al., 2009]. Careful standardization and validation of these tests is necessary.
Nonspecific parameters have been studied in the CSF to establish or exclude a bacterial etiology rapidly, including various indices of inflammation (e.g., cytokines, C-reactive protein, and lactate) and measurement of various enzyme activities [Gray et al., 1986; Lutsar et al., 1994]. With the exception of lactate, these parameters are not routinely used to assess patients with meningitis.
Contraindications
Four contraindications to lumbar punctures are as follows:
Other Tests
Most pediatric patients with bacterial meningitis are initially bacteremic, as might be expected based on the hematogenous pathogenesis of the disease. Blood cultures taken at hospital admission are valuable and are positive in 80–90 percent of cases of bacterial meningitis [Feigin et al., 1992].
Among new markers, serum procalcitonin level seems to be one of the most sensitive and specific predictors for discriminating between bacterial and aseptic infections. Preliminary experiences indicate that a serum procalcitonin level greater than 0.5 ng/mL predicts bacterial rather than aseptic meningitis in children [Dubos et al., 2008].
Brain Imaging
Neuroimaging studies, such as CT, MRI, and cranial sonography (in young infants with access through open fontanel), should be used in patients with signs of increased intracranial pressure (e.g., depressed consciousness, sluggishly reactive or dilated pupils, ophthalmoplegia, retinal changes, abnormal respiratory patterns, and cardiovascular instability). Increased intracranial pressure usually is caused by cerebral edema or other intracranial complications, such as subdural collection, thrombosis, infarction, brain abscess, and hydrocephalus [Ashwal, 1995; Tunkel and Scheld, 1995].
MRI has improved resolution compared with CT, provides additional insights into the pathogenesis of neurologic complications, and eventually may replace CT in most situations [Smith and Caldemeyer, 1999]. Cortical enhancement may be identified and is presumed to indicate cerebritis. Repeat imaging is useful in following the evolution of abnormalities [Bodino et al., 1982] and in determining the need for subsequent intervention. Abnormalities on imaging are associated with an unfavorable outcome [Packer et al., 1982]. Contrast MRI, although not usually considered a diagnostic test for meningitis, can show meningeal enhancement (Figure 80-1) [Kioumehr et al., 1995; Runge et al., 1995].
Complications
Pathophysiologic Changes
Blood–brain barrier disruption
The permeability of the blood–brain barrier increases in meningitis as a result of disrupted intercellular tight junctions and increased transendothelial pinocytosis [Quagliarello et al., 1986]. Inflammatory components, including granulocytes and matrix metalloproteinases, contribute to the increased permeability of the blood–brain barrier [Leppert et al., 2001]. This increased permeability results in increased extravasation of serum components and contributes to vasogenic brain edema, but also allows for approximately fivefold higher concentrations of antibiotics in the CSF during therapy compared with the uninfected state.
Brain edema
Brain edema during meningitis has vasogenic, cytotoxic, and interstitial components. Vasogenic cerebral edema is primarily a consequence of increased blood–brain barrier permeability (see earlier). Cytotoxic edema results from an increase in intracellular water after alterations of the cell membrane and loss of cellular homeostasis with influx of sodium and calcium into the cell. Cytotoxic mechanisms include ischemia and the effect of excitatory amino acids [Derugin et al., 2000]. Interstitial edema occurs as a result of an increase in CSF volume, through either increased CSF production (increased blood flow in the choroid plexus) or decreased resorption secondary to increased CSF outflow resistance across the arachnoid villi system of the sagittal sinus [Scheld et al., 1980]. The major dangers of extensive brain edema during meningitis are herniation of brain tissue and compression of the brainstem secondary to increased intracranial pressure, which can cause complete cessation of cerebral circulation (Table 80-2). Brain edema contributes substantially to the acute fatal outcome of bacterial meningitis [Winkler et al., 2002].
Table 80-2 Common Neurologic Sequelae after Bacterial Meningitis
Deficit | Approximate Frequency (%) |
---|---|
Hearing loss | 15–30 |
Parenchymal damage | 5–30 |
Cerebral palsy | 5–10 |
Learning disabilities | 5–20 |
Seizure disorders | <5 |
Cortical blindness | <5 |
Cerebral herniation | 3–20 |
Hydrocephalus | 2–3 |
Hyponatremia, dehydration, and inappropriate secretion of antidiuretic hormone
Patients with acute meningitis often present with hypovolemia secondary to dehydration, and some develop hyponatremia. Based on clinical signs, it is impossible to distinguish between hyponatremia secondary to dehydration and the syndrome of inappropriate antidiuretic hormone secretion (SIADH) in patients with meningitis. It also is unclear how frequently the hyponatremia is related to SIADH with secondary fluid retention [Moller et al., 2001]. SIADH results in hypotonicity of extracellular fluid, which in meningitis may contribute to cytotoxic edema [Kaplan and Feigin, 1978]. The overall risk of worsened clinical outcome owing to SIADH seems small, however, and does not justify routine fluid restrictions in patients with meningitis and hyponatremia because fluid restriction may have harmful effects on systemic blood pressure and cerebral perfusion. In cases of severe or persistent hyponatremia, SIADH should be excluded with appropriate laboratory tests.
Intracranial hypertension
Increased intracranial pressure is present early in most patients with bacterial meningitis. In infants with open fontanels and symptoms compatible with meningitis, the full fontanel may lead to the correct diagnosis. Early in the course of meningitis, cerebral hyperemia may be the most important mechanism of intracranial pressure elevation. In more advanced stages, brain edema is probably the most important factor contributing to increased intracranial pressure, but obstructive hydrocephalus, cerebritis, cerebral infarction, cerebral venous thrombosis, status epilepticus, and SIADH also contribute to increases in intracranial pressure [Brown and Feigin, 1994].
Cerebral blood flow changes
In the early phase of bacterial meningitis, an increase in cerebral blood flow is observed, which seems to be mediated by nitric oxide and oxidative radicals resulting from the inflammation in the CSF space. As the disease progresses, cerebral blood flow reductions are observed globally and focally [Pfister et al., 1992]. Global cerebral blood flow reduction is the result of reduced cerebral perfusion pressure because of increased intracranial pressure, systemic hypotension, or both, in the setting of impaired cerebral blood flow autoregulation. Focal ischemia is the result of vascular involvement of cerebral arteries and veins by the subarachnoid space inflammation [Pfister et al., 1992]. Reactive oxygen radicals and endothelins, a family of vasoactive peptides released into the CSF during bacterial meningitis, contribute to reductions of cerebral blood flow [Koedel et al., 1997; Pfister et al., 2000]. Several clinical studies have found an association between severe cerebral blood flow reduction and adverse outcome in children and adults with meningitis, making ischemia an important mediator of brain damage in meningitis [Ashwal et al., 1992; Förderreuther et al., 1992; Odio et al., 1991].
Seizures
Seizures complicate 20–30 percent of cases of childhood bacterial meningitis. Patients with severe illness are more likely to have seizures. They occur most frequently 2–3 days into the illness and cease 1–3 days later. Seizures may be focal or generalized, and the electroencephalogram (EEG) may be normal or may show generalized or focal epileptiform abnormalities, focal slowing, or only background slowing. The functional and structural alterations of the brain resulting from the meningitic process that may induce seizures include vasculitis and infarction, fever, electrolyte imbalances, and metabolic changes. Chronic seizures after recovery from meningitis are approximately 3-fold more common in patients than in age-matched control children [Bedford et al., 2001].
Deafness and Cranial Nerve Damage
Deafness is the most frequent neurologic sequela of bacterial meningitis. Of patients with bacterial meningitis, 1–30 percent develop hearing loss (see Table 80-2), and the risk is highest when meningitis is caused by S. pneumoniae. Low CSF glucose concentrations are another risk factor for hearing loss [Eisenhut et al., 2003]. Hearing impairment after meningitis can improve for several months, but many children have severe, permanent, and bilateral hearing loss [Wellman et al., 2003]. Typically, sensorineural hearing loss in both ears is found; rarely, a conductive hearing loss associated with a middle ear infection may be present. Hearing should be assessed in children with bacterial meningitis before discharge. Audiometry is an appropriate test in older children. In young infants, brainstem auditory-evoked responses or transient otoacoustic emissions can be used to detect hearing loss early [Cohen et al., 1988; Francois et al., 1997]. When hearing loss occurs, early intervention by a team that is knowledgeable in the management of this problem in infants and children should be encouraged. Cochlear implants are a consideration in the most severe cases.
Experimental studies suggest that bacteria reach the cochlea primarily via the cochlear aqueduct [Bhatt et al., 1993]. The deafness is caused by infection and inflammation in the cochlea [Klein et al., 2003]. Nitric oxide, other inflammatory mediators, and bacterial products, specifically the pneumococcal toxin pneumolysin, contribute to the loss of hairy cells in the cochlea [Amaee et al., 1995].
Neuronal Damage
The neurologic sequelae after meningitis, in addition to hearing loss (see previously), include focal sensorimotor deficits, mental retardation, seizure disorders, and cortical blindness (see Table 80-2). Detailed studies of the neurologic outcome after bacterial meningitis have led to an appreciation of the significant long-term functional impairment resulting from the disease [Grimwood et al., 1995; Bedford et al., 2001; Oostenbrink et al., 2002]. In a cohort of 5-year-old children who had survived bacterial meningitis during their first year of life, there was a 10-fold increase in the risk of moderate to severe disabilities, with 7.5 percent demonstrating learning difficulties; 8.1 percent, neuromotor disabilities; 7.3 percent, seizure disorders; 25.8 percent, hearing problems; and 11.9 percent, behavioral problems [Bedford et al., 2001]. Neurologic sequelae of childhood meningitis can persist at least into young adulthood, impairing learning during the entire time that children go to school [Grimwood et al., 2000]. S. pneumoniae consistently was associated with higher rates of disabilities than infection with H. influenzae or N. meningitidis [Grimwood et al., 1996].
Meningitis causes brain damage of cortical and subcortical structures (Figure 80-2). For much of the brain damage, particularly in the cortex, ischemia represents an important mechanism of injury (Figure 80-3) [Pfister et al., 2000]. In keeping with this notion, inhibition of excitatory amino acids and preservation of cerebral blood flow through inhibition of reactive oxygen radicals or endothelins are protective in experimental meningitis [Meli et al., 2002]. Matrix metalloproteinases, in addition to their effect on the blood–brain barrier and on cytokine release, also may contribute directly to ischemic changes in the brain.
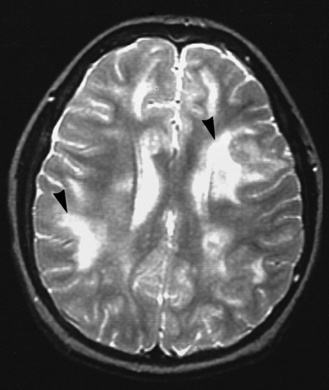
The meningitis is complicated by multiple areas of cerebritis (arrowheads and other locations).
(Courtesy of Dr. Blaine Hart.)
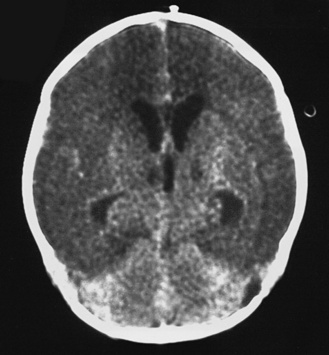
Fig. 80-3 Computed tomography scan of a 16-day-old neonate with group B streptococcal meningitis.
There is massive infarction of the anterior circulation.
(Courtesy of Dr. Blaine Hart.)
A structure frequently damaged during meningitis is the dentate gyrus of the hippocampus. This structure is important for learning and memory acquisition, and represents one of the few areas of the brain where neurogenesis is on-going throughout life. Within this structure, pathology studies in humans dying from meningitis and animal models of meningitis consistently have documented damage to neurons [Gianinazzi et al., 2003; Nau et al., 1999]. Two forms of injury seem to affect the dentate gyrus neurons [Bifrare et al., 2003]. One is mediated by activation of caspase-3 and corresponds to classic apoptosis. This form of injury preferentially affects immature, postmitotic neurons in the subgranular zone, is associated with learning deficits in experimental animals surviving meningitis, and may represent an anatomic substrate for cognitive impairment and learning disabilities after meningitis [Bifrare et al., 2003; Leib et al., 2003]. The other form of dentate gyrus injury exhibits no obvious selectivity for a subset of neuronal cells and is associated with translocation of apoptosis-inducing factor. For both forms of dentate gyrus injury, the exact molecular triggers are unknown, but ischemia does not seem to be important. Potential mediators include pneumococcal products (pneumolysin and hydrogen peroxide), inflammatory mediators (e.g., tumor necrosis factor-α), matrix metalloproteinases, excitatory amino acids, reactive oxygen species, and others [Leib et al., 1996; Meli et al., 2002].
Hydrocephalus
Meningitis leads to disturbances of CSF homeostasis, with increased CSF production and reduced CSF absorption across the sagittal sinus – arachnoid villi system [Scheld et al., 1980]. In the acute phase, these disturbances can lead to ventriculomegaly associated with intracranial hypertension. This form of ventriculomegaly is frequently transient. Chronic hydrocephalus occurs as a long-term sequela of bacterial meningitis in infants and children (see Table 80-2). Its pathogenesis is either obstructive, secondary to inflammatory changes of CSF drainage pathways, or ex vacuo, secondary to loss of brain parenchyma. Chronic internal shunting with internal drainage is necessary in patients with persistent obstructive hydrocephalus.
Septic Shock and Disseminated Intravascular Coagulation
Bacterial meningitis can be complicated by sepsis and septic shock, independent of the infecting organism. All patients with bacterial meningitis need to undergo close cardiovascular monitoring until they are clinically stabilized with antimicrobial and supportive therapy. The association of sepsis and extensive disseminated intravascular coagulation suggests infection caused by N. meningitidis [Yung and McDonald, 2003]. A similarly fulminant and devastating course can be seen with S. pneumoniae in patients without a functional spleen. These patients present with rapidly progressive signs of severe illness, rigors, severe muscular pain, and skin lesions that progress from an early maculopapular rash to petechiae to enlarging purpuric lesions [Yung and McDonald, 2003]. The organism sometimes can be visualized on Gram stain of scrapings from purpuric lesions. Signs and symptoms of meningitis often are not prominent in patients with disseminated meningococcal sepsis. Laboratory abnormalities include thrombocytopenia, hypofibrinogenemia, and massively elevated D-dimers. Overt adrenal insufficiency seems to be relatively rare in children with meningococcal disease [Riordan et al., 1999]. The most fulminant form of sepsis and disseminated intravascular coagulation is the Waterhouse–Friderichsen syndrome, which leads to sudden cardiovascular collapse associated with adrenal hemorrhage.
Extra-Axial Fluid Collections
The subdural space is a potential intracranial space situated between the arachnoid and dura. Fluid can collect in the subdural space and in the subarachnoid space. The collection in the subarachnoid space may represent loculated CSF, widening of the subarachnoid space in response to inflammation, or loss of brain parenchyma. Because imaging often cannot distinguish between a fluid collection and a widened subarachnoid space, the fluid collection as seen on imaging is referred to as an extra-axial fluid collection. Extra-axial fluid collections are found in 50 percent of children with bacterial meningitis who have cranial CT performed [Syrogiannopoulos et al., 1986].
Extra-axial fluid collections occur more commonly in bacterial meningitis during the first year of life and often are recognized during the first week of illness. The extra-axial fluid is preferentially situated over the convexities, may accumulate bilaterally, is serosanguineous with granulocytes and high protein concentrations, and is usually sterile (Figure 80-4) [Snedeker et al., 1990]. Extra-axial fluid collections often are detected on imaging studies performed because of persistent fever, clinical signs of meningitis, seizures, or focal neurologic deficits. Often, they represent a coincidental finding, but they may also be the cause of persistent increase in intracranial pressure or focal neurologic deficits. Extra-axial fluid collections, as discussed here, must be distinguished from subdural empyema, which frequently is a complication of sinusitis or otitis media and needs emergent treatment. Subdural empyema will be discussed later in this chapter.
Pathology
Subarachnoid space inflammation appears as a grayish yellow to green exudate covering the base and convexities of the brain. Histologic examination indicates that the exudate in acute bacterial meningitis consists predominantly of granulocytes; there is a mixture of lymphocytes, macrophages, and granulocytes in subacute to chronic forms of meningitis. Cerebral arteries and veins have focal infiltration of the vessel walls by the inflammatory cells. The inflammation of the vessel wall may be associated with thrombosis and vascular occlusion [Cairns and Russell, 1946]. In fatal cases of neonatal meningitis, inflammatory vasculitis is uniformly present, possibly indicating that the cerebral vasculature of the neonate is particularly susceptible to inflammatory damage [Berman and Banker, 1966]. Some of the severe structural damage in the neonatal brain after meningitis may be related to this susceptibility of the vasculature to inflammatory damage.
Damage to the brain parenchyma is manifested by signs of brain edema and frequently cerebral herniation (see later), and by areas of cerebral infarction resulting from ischemia [Arseni and Nereantiu, 1972; Berman and Banker, 1966]. Throughout the brain, there is loss of neurons, usually in a focal pattern, most extensively in patients who survived the acute meningitis for several days before succumbing to the disease. Neuronal loss is associated with a marked reaction of astrocytes and microglia.
In the dentate gyrus of the hippocampus, evidence of cell death of neurons seems common in meningitis based on limited pathologic studies in humans and extensive work in animal models [Nau et al., 1999]. Apoptotic neuronal cell death can be detected primarily in the subgranular zone of the dentate gyrus and is characterized morphologically by condensed, fragmented nuclei. The apoptotic nature of this form of cell death has been confirmed by the detection of fragmented DNA (i.e., TUNEL-stain) and activation of caspase-3 [Gianinazzi et al., 2003].
Treatment
Antibiotics
Effective eradication of the infecting pathogen requires that antibiotics display bactericidal activity in the CSF. Experimental studies have documented the fact that CSF concentrations that exceed the in vitro minimal inhibitory concentration severalfold (>10-fold for most antibiotics) are required to achieve maximal bacterial killing rates. CSF concentrations of antibiotics, even with inflamed meninges, are only a fraction of simultaneous serum concentration (usually between 5 and 25 percent), and antibiotics must be dosed higher for the treatment of meningitis than for most other infections [Täuber and Sande, 1990].
Empiric therapy should be instituted rapidly in all children with suspected bacterial meningitis. The progressive pathophysiologic alterations during meningitis, which rapidly lead to brain damage and death in most untreated patients, strongly suggest that any delay in antibiotic treatment may be harmful and should be avoided [Aronin et al., 1998, Koster-Rasmussen et al., 2008]. In practice, the appropriate sequence of obtaining CSF and blood for cultures and other tests, instituting antibiotics, and obtaining imaging studies to exclude mass lesions and other pathologies needs to be adapted to the individual patient. The sicker the patient and the more rapidly progressive the disease, the more urgent is the start of antibiotic therapy. Antibiotic therapy should not be delayed by more than approximately 15 minutes in critically ill patients for an attempt to obtain CSF or blood, despite the reduced yield of bacterial cultures if the samples are obtained after initiation of antibiotics. A CT scan is required before the lumbar puncture in all patients in whom there is suspicion of important CNS alterations, particularly if a mass lesion is suspected, and the patient has signs of increased intracranial pressure. In adults, criteria have been established to identify patients with suspected meningitis in whom a lumbar puncture can be performed safely [Hasbun et al., 2001]. Whether these criteria are valid in children has not been established. The contraindications for lumbar puncture were discussed earlier.
The choice of empiric antibiotics depends on the suspected pathogens and their antibiotic susceptibilities, which may display regional differences. All pathogens commonly encountered in a particular patient population should be covered empirically, and rare pathogens should be empirically targeted in patients with corresponding risk factors (Table 80-3 and Table 80-4). Recommended empiric treatment regimens adequate for patients without additional risk factors are shown in Table 80-5. When the causative pathogen has been isolated, and antibiotic sensitivities are known, therapy should be adjusted to cover the respective pathogen optimally (see later).
Table 80-3 Common Causes of Acute Bacterial Meningitis According to Age
Age | Pathogen |
---|---|
0–4 wk | Streptococcus agalactiae Escherichia coli Listeria monocytogenes Streptococcus pneumoniae |
1–3 mo | E. coli L. monocytogenes Neisseria meningitidis S. agalactiae S. pneumoniae Haemophilus influenzae |
3 mo to18 yr | N. meningitidis S. pneumoniae H. influenzae |
Table 80-4 Rare Causes of Bacterial Meningitis in Infants and Children
Pathogen | Predisposing Factors |
---|---|
Staphylococcus aureus | Trauma, neurosurgery, shunt |
Staphylococcus epidermidis | Trauma, neurosurgery, shunt |
Enterococcus spp. | Neonatal period |
Group A streptococcus | Pharyngitis, sepsis |
Viridans streptococci | Endocarditis, anatomic defect |
Moraxella | Otitis media |
Escherichia coli* | Urinary tract infection, immunosuppression |
Klebsiella pneumoniae | Immunosuppression |
Salmonella | Sickle cell disease, immunosuppression |
Proteus | Neonatal period, immunosuppression |
Citrobacter | Neonatal period, immunosuppression |
Other Enterobacteriaceae | Immunosuppression |
Pseudomonas aeruginosa | Immunosuppression |
Pasteurella multocida | Animal bites |
Francisella tularensis | Wild animal contacts |
Propionibacterium acnes | Shunt |
Nocardia | Chronic pulmonary disease |
* Rare past the neonatal period.
Table 80-5 Recommendations for Empiric Antibiotic Therapy of Bacterial Meningitis
Patients and Special Modifying Circumstances | Antibiotic | Dosage (IV) |
---|---|---|
Neonate/infant <3 mo | Ampicillin plus | 50–100 mg/kg every 6–8 hr |
Cefotaxime or | 100 mg/kg every 8–12 hr | |
Gentamicin | 2.5 mg/kg every 8 hr | |
Neonate preterm, low birth weight | Vancomycin plus | 15 mg/kg every 8–24 hr |
Ceftazidime | 100 mg/kg every 8–12 hr | |
>3 mo to <50 yr | Ceftriaxone or | 100 mg/kg every 24 hr (max. 4 g every 24 hr) |
Cefotaxime | 100 mg/kg every 8 hr (max. 12 g per day) | |
Drug-resistant Streptococcus pneumoniae | Ceftriaxone plus | 100 mg/kg every 24 hr (max. 4 g every 24 hr) |
Rifampicin or | 10–20 mg/kg every 24 hr (max. 600 mg every 24 hr) | |
Vancomycin | 15 mg/kg every 6 hr (max. 500 mg every 6 hr) | |
Neurosurgery, cerebrospinal fluid shunt, or head trauma | Ceftazidime plus | 100 mg/kg every 8 hr (max. 12 g per day) |
Nafcillin or Flucloxacillin | 50 mg/kg every 6 hr (max. 12 g per day) | |
(or Vancomycin plus | 15 mg/kg every 6 hr (max. 500 mg every 6 hr) | |
Aminoglycoside) | 2.0–2.5 mg/kg every 8 hr |
The duration of therapy is not strictly defined and should take into account the clinical response to treatment. Meningitis caused by N. meningitidis can be treated for 4 days [Crosswell et al., 2006], and for meningitis caused by S. pneumoniae or H. influenzae, 4–7 days treatment were not inferior to 7–14 days in a recent meta-analysis [Karageorgopoulos et al., 2009]. Meningitis caused by Enterobacteriaceae, L. monocytogenes, other unusual pathogens, or organisms with reduced antibiotic sensitivity may require longer treatment courses. Antibiotic therapy should not be prolonged past the recommended durations because of a persistent fever in a child who has otherwise displayed a favorable clinical response. Other causes of fever, such as drug fever, intravenous line infections, other undiagnosed infections, and extra-axial fluid collections, should be considered.
Streptococcus pneumoniae
For sensitive S. pneumoniae, penicillin G remains the drug of choice, and third-generation cephalosporins (ceftriaxone or cefotaxime) are effective alternatives. The prevalence of pneumococci resistant to β-lactam antibiotics and the average minimal inhibitory concentration for these antibiotics have been increasing worldwide over the last decades [Jones et al., 2004]. Penicillin and chloramphenicol are ineffective against strains displaying any degree of resistance, whereas third-generation cephalosporins may fail in highly penicillin-resistant strains. A combination of a third-generation cephalosporin with vancomycin is most commonly recommended for these strains [Bradley and Scheld, 1997]. Dexamethasone reduces CSF concentrations of vancomycin in adults, but apparently not in children. Rifampicin can be used instead of vancomycin, if the strain is sensitive. There is currently limited clinical experience with newer quinolones [Saez-Llorens et al., 2002], which look promising in experimental meningitis. In these models, the newer quinolones display strong synergism with β-lactams or vancomycin, and these combinations may provide a treatment option in cases caused by high-level resistant pneumococci [Cottagnoud and Täuber, 2004].
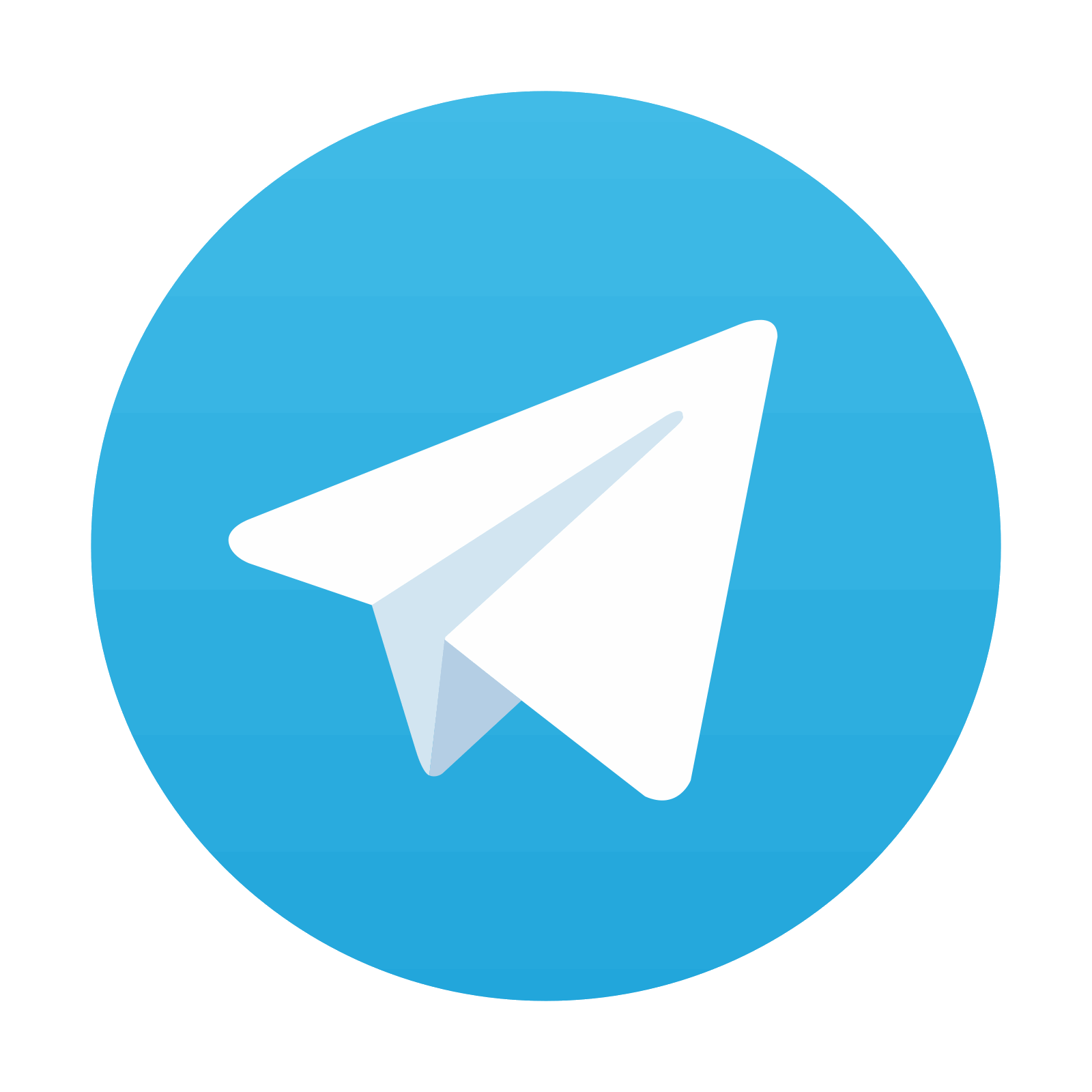
Stay updated, free articles. Join our Telegram channel
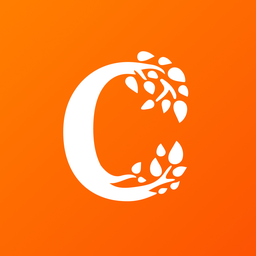
Full access? Get Clinical Tree
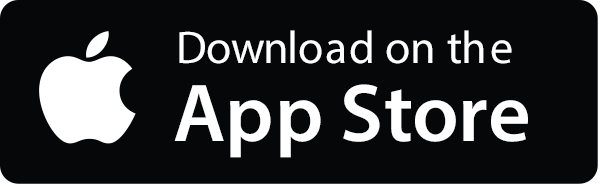
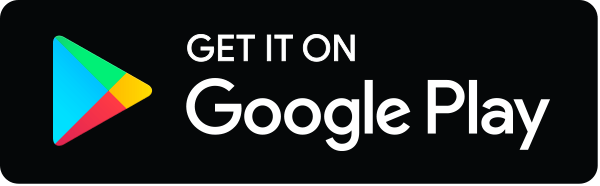