13.1 Introduction
Epileptogenesis is the process of development of epilepsy after exposure of the brain to initial precipitating injury in combination with genetic factors.1 There is typically a time gap between the exposure of the brain to these influences and development of spontaneous recurrent seizures.2 Epileptogenesis is difficult to study because patients are diagnosed after they have already developed spontaneous seizures and associated comorbidities. Based on brain regions involved, epilepsy is broadly classified into one of the following two categories: (1) generalized epilepsy, involving widespread brain regions in both hemispheres, or (2) focal epilepsy, where only select brain regions are involved. Approximately 50 million people worldwide are living with epilepsy.3
Because patients are usually diagnosed after full manifestation of epilepsy, it is difficult at the present time to prevent this disorder in humans. For this reason, we have tried treating epilepsy before it develops in rodent models as a proof-of-principle approach intended for eventual clinical translation. We succeeded in demonstrating primary prevention of a genetic form of epilepsy in an animal model. Primary prevention of epilepsy in an animal model is an important focus of this chapter, which we place in the broader context of neuroimaging epilepsy biomarkers.
Neuroimaging has a crucial role in the measurement of long-term structural and functional changes in the brains of both partial and generalized epilepsy patients noninvasively. Conventional neuroimaging methods comprise magnetic resonance imaging (MRI) morphometric analysis, proton magnetic resonance spectroscopy (1H-NMR), and positron emission tomography (PET). To measure subtle structural and functional network changes during the process of epileptogenesis, additional advanced imaging techniques, such as resting functional connectivity in functional MRI (fMRI) and diffusion tensor MRI (DTI), are being used in animal models.
To cure epilepsy, we first need to understand epileptogenesis in animal models and in patients, and then we need therapies that can prevent or reverse epileptogenesis. Antiepileptic medicines have shown promise in suppressing primary generalized but not in secondary epilepsies.4
In this chapter we review neuroimaging research strategies to identify and prevent epileptogenesis in focal epilepsy in the first section and in generalized epilepsy in the second section. We review studies from well-validated animal models of both acquired and genetic epilepsies using various neuroimaging modalities. Focal seizures discussed in this chapter include febrile seizures, models of traumatic brain injury (TBI) and posttraumatic epilepsy (PTE), kindling of temporal brain regions, such as amygdala, for TLE model and proconvulsant-induced SE model, such as pilocarpine or kainic acid, and consequent spontaneous seizures modeling temporal lobe epilepsy (TLE). Our discussion of generalized epilepsy will focus on childhood absence epilepsy. Once we understand the underlying structural and functional abnormalities in epileptogenesis in animal models,5, 6 it may be possible to implement primary prevention with treatment drugs and to track underlying structural and functional changes in human patients, as we have done successfully in a generalized epilepsy animal model.7, 8
13.2 Focal Epilepsy
13.2.1 Magnetic Resonance Imaging
MRI has been the diagnostic tool of epilepsy for many decades.9, 10 It can detect structural as well as functional changes in many type of seizures. Therefore, abnormalities on MRI have been used as a biomarker of epileptogenesis in other animal models of focal epilepsy. Febrile seizures (FSs) are a common type of seizures in children that are associated with a sudden rise in body temperature.11 The hyperthermia-induced prolonged FSs model in rat shows that T2 signals from serial MRIs before and after seizure onset were increased in the dorsal hippocampus, piriform cortex, and amygdala by 75% and 87.5% of these rats one and eight days after seizures, respectively.12 In another rat study, hippocampal T2 signal increased at 1 month13 after the induction of FSs. These abnormal T2 signals have an increased likelihood of developing spontaneous seizures,12, 13 but itself was not found to be a predictor of epileptogenesis13 (Figure 13.1). Febrile status epilepticus (FSE) shows reduced T2 relaxation times in amygdala on high-magnetic-field MRI hours after FSE and predicted experimental TLE.14 These reduced T2 signals in temporal lobe structures, like amygdala, signify injury-sensor high-mobility group box 1, a trigger of inflammatory cascades, and indicate underlying changes in neuronal integrity that promote epileptogenesis and provide early markers for subsequent TLE development.14
Figure 13.1. T2-weighted MR imaging signal intensities after prolonged experimental febrile seizures (FSs) in rat. (A) The control and the seizure animal groups were imaged before the seizures induction on postnatal day 10, P10, to provide baseline intensities, and on P12 (24 hours after seizure induction, seizure group) as well as 8 days after the seizures. Regions used for analysis are delineated in white in baseline image. Increased signal intensity can be appreciated in the dorsal hippocampus (white arrows), amygdala (asterisk), and piriform cortex (white arrowheads). (B) T2-weighted signal intensity modulation by prolonged experimental FSs: (i) a significant increase of T2-weighted signal occurred in dorsal hippocampus, by 8 days after the seizures, (ii) in piriform cortex, signal intensity increased significantly at 24 hours and 8 days after seizure, (iii) in the medial ventroposterior thalamic nucleus, signal intensity increased significantly at 8 days and there was a strong trend for increased signal at 24 hours (diamond) after seizure.
PTE is another type of partial epilepsy that results from an insult to the brain from an external mechanical force, leading to TBI with permanent damage to brain cells. Periods of increased abnormal plasticity occur in the process of posttraumatic epileptogenesis. This change mimics the critical period of development.15, 16 Quantitative T2 image signal evaluation has been used to analyze the severity of cortical damage and neural and motor impairment in a lateral fluid percussion injury (LFPI) rat model of TBI, and T2 signal intensities are correlated with motor deficits and histological lesion volume and also differentiate moderate from severe TBI.17 Conventional and advanced quantitative MRI changes, such as T2, T1ρ, and diffusion tensor metrics acquired after trauma in the LFPI rat model, predict functional and pathological changes several months after trauma.18 These results show that early changes in the hippocampus and perifocal brain regions may alert us to long-term brain changes after trauma.
In lithium-pilocarpine-induced status epilepticus (SE), rats without MRI abnormalities or altered T2 relaxation times did not end up developing epilepsy, whereas those with increases in T2 relaxation times and abnormalities in the T2-weighted images, specifically at the level of piriform and entorhinal cortices, subsequently developed epilepsy at 4 months after induction.19 Apart from these findings, other studies have also documented more widespread T2 signal decreases in the acute stages after seizure induction and associated with spontaneous seizures in a later stage.20 Acute MRI changes in the parietal cortex, hippocampus, piriform cortex, and thalamus after lithium-pilocarpine-induced seizure have been shown to be predictive of later hippocampal damage.21 These findings suggest temporal intricacies in T2 signal changes post-SE partial epilepsy models likely predict the evolving processes of epileptogenesis.20, 22 Similar to chemical-induced SE models, SE induced by electrical stimulation of the rat amygdala resulted in increased T2 signal intensity in the amygdala beginning 2 days after SE and pathologic T2 was observed in the piriform cortex, midline thalamus, and hippocampus. These initial MRI changes showed a low predictive value for the subsequent severity of epilepsy,23 and acutely increased T2 signal intensity in the hippocampus, results in acquired epileptogenesis in limbic system.24 These MRI signal changes have the potential to become a clinically useful biomarker after careful further study.
13.2.2 MRI Morphometric Analysis
Conventional MRI can delineate different brain structures on the basis of gray and white matter structures within the brain. MRI morphometry and volumetry take advantage of this property of MRI for image analysis to compare the size and shape of various brain structures. Voxel-based morphometry (VBM) is performed by spatially normalizing MRI images across all the subjects in the study into the same stereotactic space, segmenting the gray matter from the spatially normalized images and smoothing the gray matter segments, and then performing voxel-wise statistical comparison of the smoothed gray matter images from the two groups to produce a parametric map of structural regions.25 Initial studies using VBM analysis included a study of hippocampi of London taxi drivers showing that the posterior hippocampi of taxi drivers were larger than those of control participants.26 VBM analysis has been used to study epilepsy and other brain disorders. Volumetry is another forms of analysis where the volumes of regions of interest are calculated for comparison. VBM and volumetric changes in the limbic structures like CA1 and dentate gyrus in the hippocampus have been reported in SE21, 24, 27 as well as in the LFPI model of PTE.28–31 These techniques may play a role in assessing structural changes in the cortical and subcortical structures compromised in epileptogenesis.
13.2.3 Proton Magnetic Resonance Spectroscopy
1H NMR is a technique for identification and quantitation of the metabolites in healthy and brain with various diseases.32, 33 Major metabolites detected by this technique are N-acetylaspartate (NAA), creatine (Cr), phospholipids with choline (Cho), lactate (Lac), myo-inositol (mIns), and glutathione (GSH). NAA is a marker of neuronal and axonal change; Cr and/or phosphocreatine show brain energy metabolism; Cho shows membrane turnover, inflammation, or demyelination; Lac is a marker of anaerobic glycolysis;33 mIns and GSH are considered important markers of inflammation.34 Imbalance of excitatory and inhibitory neurotransmitters like glutamate, glutamine, and gamma-aminobutyric acid (GABA) likely show the process of epileptogenesis.35, 36 Acute and chronic reductions in NAA35, 37 and reductions in excitatory and inhibitory neurotransmitters38 have been shown in the post-SE rat model of TLE. Increases in mIns and GSH have been shown after seizure induction in rats.35 The above findings suggest capability of PMRS in identifying biomarkers associated with structural and/or functional changes occurring in the epileptogenic process.
13.2.4 Positron Emission Tomography
PET measures emissions from a large number of metabolically active radiotracers. PET visualizes distribution of the tracer by visualizing receptor density in the brain and identifies pathology with altered metabolism in epilepsy. For the same reason, PET was applied to epilepsy research several years after it was first developed.39 Recent studies in the field show many radiotracers are helpful in identifying epileptogenesis in rodent models of epilepsies.40–44 The rat model of TLE shows severe glucose hypometabolism associated with epileptogenesis.45
13.2.5 Functional Magnetic Resonance Imaging
Functional MRI uses paramagnetic properties of deoxyhemoglobin in the blood to generate MRI signal. Because the level of deoxyhemoglobin changes with brain function, this property of hemoglobin was used to develop a new MRI image contrast named blood-oxygen-level-dependent (BOLD) fMRI by Ogawa and colleagues.46 Since EEG detects location and magnitude of brain activity, EEG-correlated fMRI has been used to investigate epileptogenic networks in focal epilepsy.47, 48 Epilepsy is known to involve abnormal neural networks,47 and fMRI can detect neuronal networks involved during seizures. The chemical-induced seizure model shows robust fMRI changes correlated with seizure.49 The above-mentioned studies paved the way to use fMRI as a biomarker to track disease progression and treatment efficacy in focal epilepsy.
13.2.6 fMRI with Resting Functional Connectivity
Functional connectivity is an fMRI technique where temporal correlation of a neurophysiological signal is measured from remotely located brain areas.50 Biswal and colleagues demonstrated correlation of low frequency (<0.1 Hz) changes in the primary sensory cortex induced by hand movement both within and between hemispheres.51 In our recent study of the LFPI rat model of PTE, we reported decreased connectivity between the ipsilateral and contralateral parietal cortex and between the parietal cortex and hippocampus on the side of injury as compared to sham-operated animals (Figure 13.2)6 four months after induction of injury. These injured animals have increased seizure susceptibility evaluated by the pentylenetetrazole test. Injured animals also show abnormal negative connectivity between the ipsilateral and contralateral parietal cortex. This is the first evidence on decreased functional connectivity after experimental TBI.6 These abnormal connectivity changes can serve as a useful biomarker for identifying individuals with a risk of developing PTE and may be useful for monitoring prevention of epileptogenesis by early treatment.
Figure 13.2. Decreased resting functional connectivity in TBI rats vs. sham-operation control rats. Two coronal MRI slices (A, B) from a rat with lateral fluid percussion injury (FPI) (note the tissue loss in the left hemisphere) demonstrating the brain regions of interest (ROIs) that were used on coronal BOLD-fMRI images for resting BOLD-fMRI signal correlation analysis. Four ROIs were made in each hemisphere (8 ROIs total): frontal cortex, parietal cortex, hippocampus, and thalamus (L, left; R, right). Panel B shows schematically using arrows the significant differences in connectivity (1) between the ipsilateral and contralateral parietal cortices and (2) between the ipsilateral parietal cortex and hippocampus in the rats with lateral FPI and control rats. Statistical significance: *p < .05. (C) Example of resting functional connectivity in a control rat. Pearson correlation values are shown using the left parietal cortex ROI (B) correlated to the whole brain. Some positive correlation is seen with the contralateral parietal cortex and ipsilateral hippocampus. (D) Example of the same analysis for the left parietal cortex ROI in a TBI animal shows reduced connectivity. Warm colors represent increases and cool colors decreases in resting-state connectivity; scale bars are for Pearson correlation with display threshold = 0.2. Voxels lying outside the brain or in CSF are not shown.
13.2.7 Diffusion Imaging
Diffusion-weighted imaging (DWI) measures the magnitude of diffusivity of water protons, whereas DTI measures both the magnitude and the directionality.52 Water diffusion is hindered by extracellular as well as intracellular microstructures, and therefore these techniques can detect subtle changes in the pathological brain5, 53, 54 where magnitude and directionality of water diffusion is altered. Apparent diffusion coefficient (ADC) is an average measure of magnitude of water diffusion in biological tissue. One of the several metrics of DTI is fractional anisotropy (FA), which measures the degree of anisotropy of water diffusion in a tissue under observation and reflects alignment of cellular structures within the tissue like white matter fiber tracts5, 54 and other cellular structures that simulate white matter fibers.55 It has a value of 0 for least anisotropic tissue and 1 for most anisotropic.52, 56 In an animal model of PTE, diffusion values have been predictive of increased seizure susceptibility.29 The FPI-induced rat model of moderate TBI shows that hippocampal DWI correlate with EEG parameters and with mossy fiber sprouting density in the ipsilateral hippocampus at early as well as chronic time points after TBI.29 ADC changes are also correlated with seizure susceptibility in FPI model of PTE.29, 57
White matter fiber tractography is another DTI method of generating a map of connection of white matter fibers traveling from one to another direction in the brain by using diffusion images acquired in multiple directions. This technique uses known white matter fiber bundle as a starting point, which allows assessment of white matter integrity neighboring to that start point called seed region. Decreased ADC and increased FA have been reported to be associated with microstructural alterations in the hippocampus of rats after FSs.58 The rat model of status epilepticus induced by pilocarpine shows that DWI is a sensitive technique for the early identification of seizure-induced neuronal cell damage.59 A decrease in FA was seen in multiple brain areas and was associated with altered tissue microstructure in the kainic-acid-induced rat model of epilepsy.60
All the above studies show that DWI and DTI measurements are sensitive in detecting epilepsy-related changes during epileptogenesis.61
13.3 Generalized Epilepsy
In this section we discuss imaging findings in generalized epilepsy focusing on childhood absence epilepsy (CAE). Widespread cortical and subcortical network impairment leads to transient altered consciousness in absence seizures. Chronic resting-state dysfunction in the cortical and subcortical network may lead to interictal psychosocial comorbidities in absence epilepsy.
Early and effective seizure treatment in rodent models and possibly human patients can have long-term beneficial effects on both seizures and epilepsy comorbidities. Early and sustained treatment of seizures with ethosuximide (ESX) in a rodent absence epilepsy model before seizure onset led to markedly reduced seizures, which continued for at least 3 months after termination of treatment68 (Figure 13.3). Subsequent work examined developmental changes in network connectivity related to epileptogenesis5, 6, 69 as well as activity-dependent changes in ion channel expression during the course of epileptogenesis.68, 70, 71 We found that early treatment in at least two rodent absence models and with antiseizure medication led to beneficial effects not only on seizures, but also on epileptogenesis-related structural and molecular changes as well as behavioral comorbidities.7, 8, 72
Figure 13.3. Early ethosuximide (ESX) treatment persistently suppressed the development of spike-wave discharges (SWDs) in WAG/Rij rats, even after stopping treatment. (A) Epileptic WAG/Rij rats were given either normal drinking water (H2O group), ESX from age p21 through age 5 months and then normal drinking water from age 5 to 8 months (ESX 4-month group), or ESX continuously from age p21 through 8 months (ESX continuous group). EEG was recorded 1 day before stopping ESX and 1, 14, 30, 60, and 90 days after stopping ESX. (B) Quantification of effects of early ESX treatment on percentage time in SWD. Even after stopping ESX, percentage time in SWD remained markedly reduced in the treated rats (ESX 4-month group) when comparing all time points for days 1 through 90 to rats on normal H2O.
Effective treatment with ESX may also have a beneficial disease-modifying effect in human patients with absence epilepsy, based on improved long-term seizure remission off medications.73 With better genetic understanding of epilepsy it will hopefully soon be possible to initiate treatment prior to disease onset with the goal of improving long-term outcome.
Above results are suggestive of a “critical period” early in development during which treatment can block both molecular and electrophysiological symptoms of epileptogenesis. With the help of noninvasive biomarkers we can monitor progression of epileptogenesis severity and efficacy of therapy after a particular treatment.
13.3.1 MRI Morphometric Analysis
VBM can reveal subtle structural changes, when conventional MRI scans reveal normal anatomy. VBM show gray matter abnormalities in the thalamus and frontal cortex, supporting the role of the thalamocortical network in the mechanisms of generalized seizures.74 VBM should be checked for consistency in user-specific parameters like type and level of statistical correction, modulation, smoothing kernel, adjustment for brain size, subgroup analysis and software version before it can be used clinically as a biomarker.75, 76
13.3.2 Functional MRI
fMRI studies in rat models of genetic absence epilepsy report BOLD fMRI signal abnormalities during spontaneous spike-wake discharges.47, 77, 78 Increases were most prominent in focal regions of somatosensory cortex, motor cortex and thalamus, while the occipital region was spared.78–80 These regions were shown to have both increased neuronal firing and increased CBF during SWD.78, 80 There are some inconsistencies in findings like BOLD fMRI increase versus decrease81 and anesthesia among some limitations.47 In spite of these variations, fMRI has been proved to be useful in identifying functional abnormalities in epileptogenesis.
fMRI studies of bicuculline-induced generalized tonic-clonic seizures show that BOLD fMRI increases were still largest in somatosensory cortex, while decreases were seen in the hippocampus.77, 82, 83
Blumenfeld and colleagues have performed simultaneous EEG, fMRI, and behavioral testing during typical childhood absence seizures to demonstrate that seizures with impaired consciousness exhibit widespread bilateral changes in cortical and thalamic networks.62, 63 In the interictal period, children with absence epilepsy exhibit heightened features of attention impairment, anxiety, and depression,64, 65 which may be related to long-term alterations in resting network functional connectivity.66, 67 Studies of CAE patients show fMRI increases in the bilateral thalamus and occipital cortex, while decreased fMRI signals were observed in the bilateral lateral parietal cortex, precuneus, cingulate gyrus, and basal ganglia.62, 66, 84–86 The fMRI time course signal changes of childhood absence seizure show small early fMRI increases in the medial/lateral parietal cortex and orbital/medial frontal >5 seconds before seizure onset, followed by intense fMRI decreases continuing up to 20 s after seizure offset.63 This dynamic pattern of fMRI signal changes shows the temporal complexity of seizure-related fMRI changes. These changes were not detected by hemodynamic response function modeling. These studies show that identification of a seizure network via these fMRI changes provides potential targets for therapy, and for monitoring of therapeutic efficacy.
13.3.3 Functional MRI with Resting Functional Connectivity
fMRI-based resting functional connectivity is a noninvasive method for assessing brain network connectivity.87, 88 This technique can easily be performed and translated from animal studies to use in human epilepsy patients. Resting functional connectivity is useful in studying long-range interactions. Since generalized epilepsy such as absence seizure involves the thalamocortical network, it may be a robust method for measuring long-term changes, even when seizures are not occurring. It will also be useful to assess network changes during treatment period when seizures are blocked by medication.
In WAG/Rij rats, we observed increased cortical-cortical correlations at rest, when no seizures were present.69 These effects were not present in nonepileptic controls (Figure 13.4). We see strongest connectivity between regions most intensely involved in seizures, predominantly in the bilateral somatosensory and adjacent cortices. Group statistics revealed higher resting interhemispheric cortical-cortical correlations in WAG/Rij rats compared to nonepileptic controls (Figure 13.4). Our findings suggest that activity-dependent plasticity may lead to long-term changes in epileptic networks that can be detected even at rest. With the help of further studies, we should be able to determine whether preventing epileptogenesis, with antiepileptic drugs, will also prevent the above-mentioned abnormal functional connectivity in epileptic networks in this rat model.8 A study of human absence epilepsy children showed significantly increased interhemispheric correlation between orbitofrontal cortex regions.66 The above-mentioned studies showed that abnormalities were observed in both animal model and human CAE patients during resting state. Therefore, these findings have potential to serve as an interictal biomarker of seizure severity in CAE and possibly in other generalized epilepsies.
Figure 13.4. Resting functional connectivity differences in epileptic WAG/Rij rats versus Wistar control rats. (A) Examples of left and right mean fMRI signal time courses in reference regions at rest when SWDs are not occurring. WAG/Rij rat (A) shows high correlation between slow (<0.1 Hz) changes in left and right reference regions. Nonepileptic Wistar control rat (B) shows less interhemispheric correlation in left and right reference regions. (C) WAG/Rij rats show increased connectivity in contralateral somatosensory and adjacent cortices. Left cortex reference region of interest versus whole brain. Increases are in warm colors and decreases are in cold colors. Very similar results were obtained using the right cortex ROI as reference. Wistar control rats show only localized connectivity in the ipsilateral (left) somatosensory cortex (data not shown). (D) Interhemispheric resting-state cortical connectivity. Increased interhemispheric resting cortical connectivity in WAG/Rij rats (mean ± standard deviation of z scores, from left and right brain regions) compared to nonepileptic Wistar controls. **p = .01, one-way ANOVA followed by Tukey’s HSD method for post hoc pairwise comparisons. Results were very similar using either the left ROI time course as reference (red) and calculating mean z score for all voxels in the right ROI, or using the right ROI time course as reference (blue).
13.3.4 Diffusion Tensor Imaging
DTI measures anisotropy of water diffusion in biological systems. Water diffusion is highly anisotropic in white matter structures56 in the nervous system because of its hindrance in the perpendicular direction by the axonal membrane and myelin sheath, which jointly modulate the degree of anisotropy. White matter microstructures are altered in epilepsy; for instance, infantile spasm, a form of pediatric epilepsy, has been associated with abnormal myelination.89, 90 Therefore, DTI detects subtle white matter changes in epilepsy and other disorders where white matter is affected.91–93
We studied beneficial effects of chronic and early pharmacological treatment with ESX on epileptogenesis in two genetic absence epilepsy models. In our study of WAG/Rij rat model of absence seizures, we showed reduced FA and increased perpendicular diffusivity in the anterior corpus callosum, which was absent in young rats before seizure manifestation or in nonepileptic controls5 (Figure 13.5A). Genetic Absence Epilepsy Rats from Strasbourg (GAERS) show more pronounced reduced FA and increased perpendicular diffusivity in anterior corpus callosum and internal capsule.5 The white matter structural changes we see can be due to a reduction in myelin94, 95 and/or decreased axon fiber density.96, 97 They may also be due to changes in the density and orientation of crossing fibers in pathways connecting regions of seizure activity.
Figure 13.5. Prevention of epileptogenesis in WAG/Rij rats. (A) Epileptic adult WAG/Rij (8 months) and GAERS (1.7 months) rats, but not young WAG/Rij rats (1.7 months), have decreased fractional anisotropy (FA) in anterior corpus callosum compared to controls. T-maps at −1.3 mm from bregma are shown, with warm colors representing decreased FA when compared with nonepileptic controls, and cool colors represent increased FA. (i) In adult epileptic WAG/Rij rats, decreased FA was observed in the anterior corpus callosum (CC). (ii) In young WAG/Rij rats, anterior corpus callosum does not show decreased FA when compared with controls. (iii) In adult epileptic GAERS, extensive decreased FA was observed in the anterior corpus callosum. Unlike the WAG/Rij rats, the fornix showed increased FA in GAERS when compared with controls, which may represent a strain difference not directly related to seizures. t value threshold = 2.00, extent threshold = 50 voxels, and FA threshold = 0.30. (B) Chronic ESX treatment improves white matter FA based on midline CC and in the internal capsule (IC) compared to age-matched. Image is horizontal slice extending from AP +3.6 mm to −5.6 mm from bregma. (C) Midline corpus callosum shows significantly increased FA, and a trend in decreased perpendicular diffusivity (λ) in ESX treated WAG/Rij rats compared to untreated control WAG/Rij rats, with no change in parallel diffusivity (λ∥). (D) Internal capsule also exhibited significantly increased FA, along with significantly decreased λ in ESX treated WAG/Rij rats compared to untreated controls, with no change in λ∥.
In our recent study we showed antiepileptogenesis in WAG/Rij rats. These rats received either ESX for 2 months (postnatal months 2–3 or 4–5) or ESX for 4 months2–5 in their drinking water. Control rats drank plain water. We measured EEG during treatment, and 6 days and 2 months posttreatment. A behavioral test was performed 6 days posttreatment. Ex vivo DTI was performed after treatment. SWD were suppressed during treatment, 6 days, and 2 months posttreatment in the 4-month group. Duration of afterdischarges elicited by cortical electrical stimulation 6 days posttreatment was also suppressed. Increased DTI metrics FA in corpus callosum and internal capsule were found7 (Figure 13.5B–C). We didn’t see large effects on any parameters after shorter treatments with ESX. Finally, we showed widespread effects of chronic ESX treatment not only within but also outside the circuitry in which SWD are initiated and generated, including preventing epileptogenesis and reducing depressive-like symptoms. Our findings suggest that chronic ESX treatment of seizures in the cortex in absence epilepsy may lead to recovery of microstructural changes in white matter pathways during repeated seizure discharges.
The animal models will enable histological studies to determine microstructural mechanisms for changes observed on DTI. In addition, DTI can also be a potential imaging biomarker for monitoring treatment by showing structural changes in affected brain regions in epilepsy.
13.4 Conclusions
The prevention of epileptogenesis has been shown in a few animal models of generalized epilepsy, whereas the process of epileptogenesis has been studied in numerous additional animal models. Advanced neuroimaging methods enable the detection of subtle changes in brain networks during epileptogenesis by using network-based methods such as DTI, fMRI, and fMRI with resting functional connectivity, which are being further developed and applied to understand clinical cases in human epilepsy. Use of these imaging techniques as biomarkers of epileptogenesis may ultimately help in studying the prevention of epileptogenesis in people with epilepsy, leading to a marked improvement in clinical outcome and quality of life.
Acknowledgments
This work was supported by grant NIH R01 NS049307, by the Epilepsy Foundation Award ID 123505 (AMM), and by the Betsy and Jonathan Blattmachr family.
References


















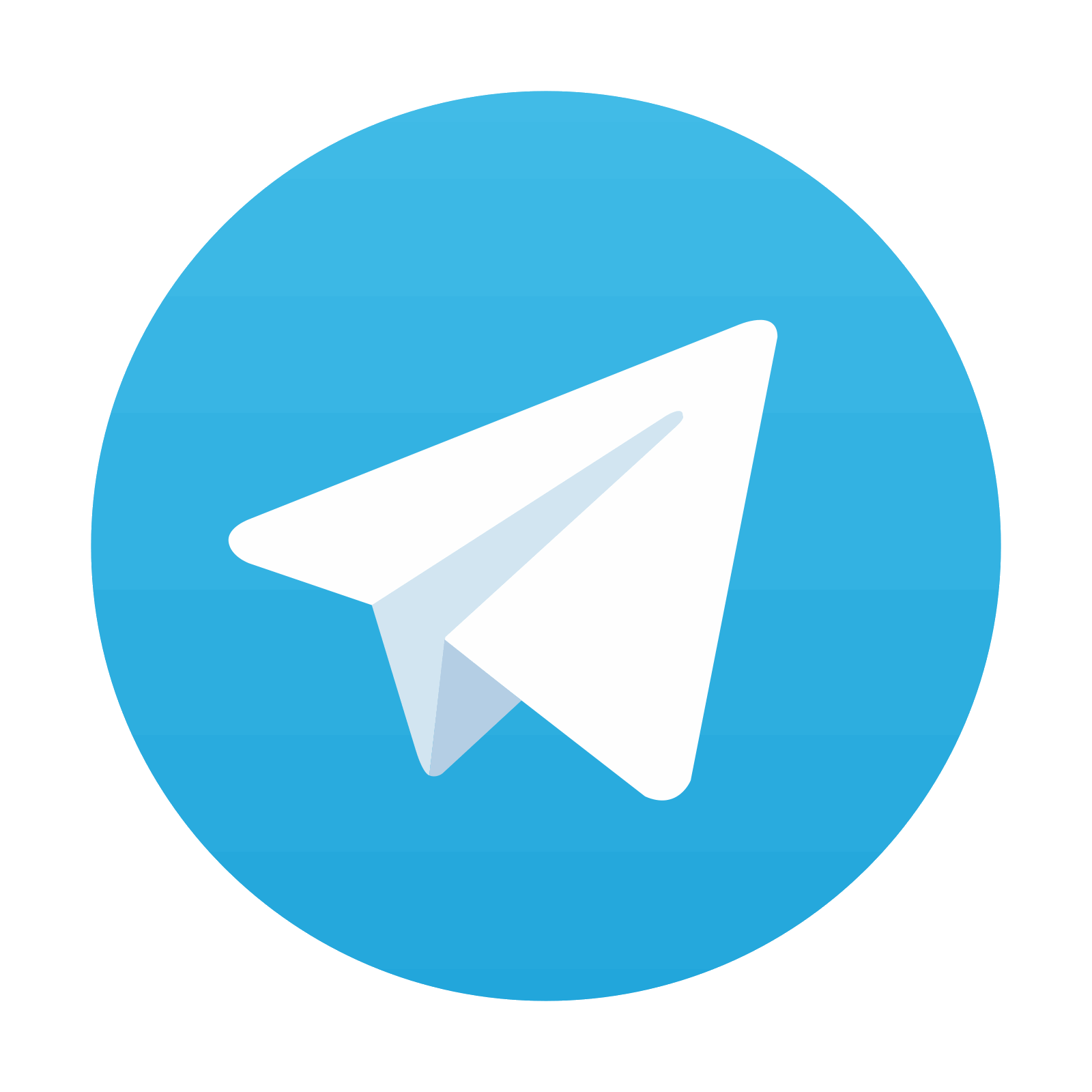
Stay updated, free articles. Join our Telegram channel
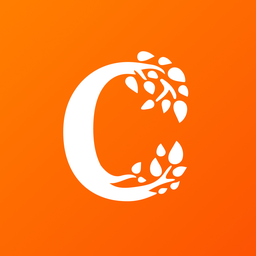
Full access? Get Clinical Tree
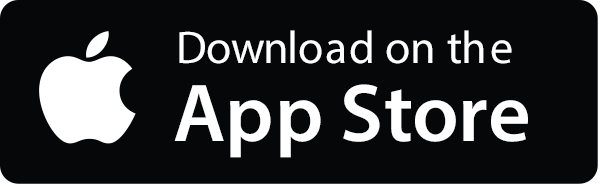
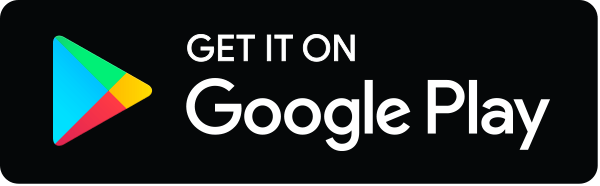
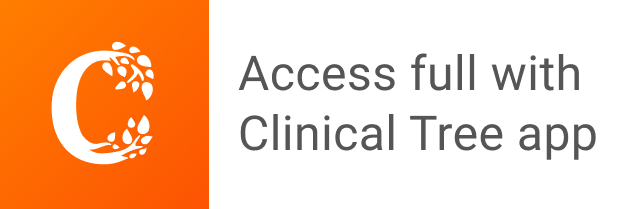