3.1 Introduction
Between 30% and 49% of all epilepsies are secondary to acute or chronic brain diseases (Figures 3.1 and 3.2).1, 2 This number is even higher in elderly patients, who most commonly develop seizures after cerebrovascular or neurodegenerative disorders. In contrast, young adults are more likely to acquire epilepsy due to traumatic brain injury or tumors or multiple sclerosis (Figure 3.2). In this chapter we focus on imaging biomarkers of acquired epilepsies in humans as a consequence of stroke, trauma, brain tumors, multiple sclerosis, and Alzheimer’s disease (Table 3.1).
Figure 3.2. Relative risk of unprovoked seizures after brain insults.
Table 3.1 Evidence Levels of Imaging Biomarkers in Acquired Epilepsies
Imaging biomarker | Evidence level |
---|---|
Stroke | |
Cortical location | ++ |
Location in anterior circulation/MCA territory | ++ |
Parietotemporal lesions | (+) |
Lesion size | ++ |
Primary or secondary hemorrhage | ++ |
Lobar brain microbleeds | (+) |
Increased 55Co uptake | (+) |
Decreased regional cerebral blood flow and oxygen consumption | (+) |
Traumatic brain injury | |
Focal lesions on initial brain scan | ++ |
Intracerebral hemorrhage | ++ |
Hemosiderin deposits surrounded by gliotic wall | + |
Lesions requiring surgical treatment | + |
Depressed skull fractures | + |
Hydrocephalus | (+) |
Open skull injury with brain prolapse | (+) |
MT > T2 mismatch | (+) |
Blood-brain barrier dysfunction | + |
Brain tumors | |
Visual tumor grading | ++ |
Frontal, temporal, or parietal tumor location | + |
Cortical involvement | + |
Tumor size | ++ |
Hemosiderin deposits | + |
Multiple sclerosis | |
Intracortical lesions | ++ |
Alzheimer’s disease | |
Hippocampal atrophy | (+) |
3.1.1 Acute and Remote Symptomatic Seizures
Two types of seizures after acute brain insults need to be distinguished.3 Acute symptomatic seizures, also termed early-onset seizures, occur in close temporal relationship to an acute brain disease and are directly provoked by neuronal, metabolic, toxic, or other conditions. Typically, seizures are considered acute symptomatic if they occur within 7 days of cerebrovascular disease or trauma; however, longer intervals might be appropriate for tumors or hemorrhages.
In contrast, remote symptomatic seizures, commonly named late-onset seizures, occur as a consequence of a distant brain insult and are considered to be unprovoked. By definition, the latent period between an insult and the seizure needs to be at least 7 days. The discrimination of early- and late-onset seizures is paramount because both entities differ in pathophysiological mechanisms, risk factors, recurrence rates, and outcome. There are also significant distinctions in biomarkers between acute and remote symptomatic seizures.
The recurrence rate significantly differs between acute (19%) and remote (65%) symptomatic seizures.4 Seizure recurrence is particularly common in patients with a first late-onset seizure after stroke or traumatic brain injury with rates ranging from 70% to 90%.4–8 These frequencies are similar to those observed in nonlesional patients after two unprovoked seizures. However, according to the new practical definition of epilepsy, the clinician does not have to wait for a second seizure to occur to diagnose epilepsy and can do this after a first seizure if the recurrence risk is higher than 60% within 10 years.9 Hence, a patient with a single late-onset seizure after stroke can be defined as having epilepsy and antiepileptic treatment might be initiated.
3.1.2 Biomarkers of Epileptogenesis
Several months or years may pass between a brain insult and the occurrence of seizures. This latent period might extend to 20 years in gradually developing neurodegenerative disorders. Epileptogenesis being a slow process, there is plenty of time for intervention. However, most medical trials to prevent epileptogenesis were unsuccessful.10
One of the results of these failures is the lack of a suitable biomarker to select patients at risk of developing epilepsy, to specifically target treatments for this enhanced population, and to use this biomarker to monitor treatment outcomes. The specific clinical question to be asked is, “Which patients are likely to develop recurrent seizures after a brain insult, and which of them might benefit from medical treatment?”
Imaging will play an important role to provide answers to this problem. However, there is still a long way to go. Currently, imaging can identify risk factors for acquired epilepsies, but these would not yet qualify as valid biomarkers. In the future, pathophysiological knowledge on epileptogenesis will need to be translated from experimental and animal studies into clinical imaging research to provide more insights into the development of symptomatic epilepsy.
3.2 Stroke
3.2.1 Epidemiology
Stroke is the most common cause of seizures and epilepsy in the elderly,11 mainly due to the high prevalence of stroke in this population. Nevertheless, seizures are not a very common consequence of stroke. The incidence of both acute (≤7 days) and remote (>7 days) symptomatic seizures ranges between 2% and 6%.12 The frequency of recurrent seizures is approximately 2% to 4%.12 The incidence of acute and remote symptomatic seizures after childhood stroke ranges from 20% to 25% and is markedly higher compared to adults.13
The frequency of seizures and epilepsy after stroke varies from one subtype to another.14–16 The highest incidence is observed in venous infarction, followed by intracerebral hemorrhage and cerebral infarction with hemorrhagic transformation. Primary ischemic stroke without hemorrhage has the lowest incidence of seizures.
The majority of acute symptomatic seizures occur within the first 24 hours after stroke.17 They are an important risk factor for delayed seizures,6, 18 and these are most likely to occur within the first year after stroke.17
3.2.2 Imaging Biomarkers
Imaging plays an important role in determining the risk of poststroke seizures and is needed to establish the location and size of the lesion, stroke subtype, and presence of hemorrhage.
3.2.2.1 Stroke Location
Cortical lesion location is the best characterized risk factor for seizures and epilepsy after stroke. This result is supported by a variety of studies with different designs and applies to both ischemic and hemorrhagic stroke.15, 18–21 However, it remains controversial whether seizures also occur after subcortical stroke. Some studies reported infrequent seizures after subcortical stroke,22–25 whereas others did not find an association.26, 27 Possibly, some of the patients with seizures after lacunar infarcts also had cortical involvement not evident on CT scans. On the other hand, subcortical stroke could also provoke seizures through the neurotoxic effects of acute glutamate release, reduction of cortical inhibition due to diaschisis, or disruption of thalamocortical loops.
Poststroke seizures are also associated with infarcts of the anterior circulation6, 23, 28 and, more specifically, of the medial cerebral artery territory.16, 29 Conversely, seizures are unlikely in posterior circulation stroke, especially if only infratentorial structures are affected. Only few studies analyzed the association of stroke location with seizures in more detail. Some found an association on a lobar level with parietotemporal cortical lesions.30–32 Others did not find a correlation at all.33 Although a detailed voxel-based lesion symptom mapping study was not yet completed, these results argue against a specific cerebral region susceptible to generating seizures after stroke. The findings rather suggest that seizures can occur after disruption of any part of the cerebral cortex without a preference to a specific location.
3.2.2.2 Lesion Size
Several studies found an association of poststroke seizures with size of the infarct.17, 18, 20, 29 A volumetric analysis showed a mean lesion volume in patients with and without seizures of 4 ml and 16 ml, respectively34 (Figure 3.3). A lesion size ≥ 70 ml increased the odds ratio of seizures fourfold. Conversely, the authors also demonstrate that although seizures are more likely in large lesions, also small infarcts can cause seizures if the cerebral cortex is strategically affected.
Figure 3.3. Lesion volume in patients with and without (A) acute symptomatic or (B) remote symptomatic seizures after stroke.
3.2.2.3 Intracerebral Hemorrhage
Remote symptomatic seizures in patients with intracerebral hemorrhage were recently shown to be independently associated with lobar brain microbleeds.21 Lobar microbleeds are commonly a manifestation of an underlying vasculopathy, i.e., cerebral amyloid angiopathy. Hence, these results suggest an increased risk of late-onset seizures in patients with intracerebral hemorrhage due to cerebral amyloid angiopathy. However, further studies will be needed to demonstrate a direct correlation of seizures with amyloid angiopathy.
3.2.2.4 Functional Imaging Studies
Few functional imaging studies have compared stroke patients with and without poststroke seizures. Two 15O2 PET studies showed a decreased regional cerebral blood flow (rCBF) and oxygen consumption (rCMRO2) in stroke patients with seizures.35, 36 The authors concluded that a more severe brain ischemia was associated with poststroke seizures. However, one cannot differentiate whether these functional changes are a cause rather than consequence of the seizures.
In another study, increased binding of cobalt-55 (55Co) was demonstrated in 60% of patients with remote symptomatic seizures after stroke, whereas no increases were seen in stroke patients without seizures.37 The degree of 55Co uptake is known to correlate with inflammatory response and the severity of ischemic damage within the prior 2 months. Hence, the authors interpret these findings as evidence for recurrent stroke as a cause for poststroke seizures. However, a more likely explanation is that increased 55Co uptake corresponds to excitotoxic damage after seizures, and is not a cause but a consequence of epilepsy.
3.2.2.5 Other Biomarkers
Clinical biomarkers most commonly shown to be associated with seizures after cerebral infarcts are stroke severity and etiology. Some authors have demonstrated that severe strokes correlate with seizures.17, 19, 33 Others argue that increased stroke severity is only a manifestation of bigger lesion size and cortical infarct location in patients with seizures.38
Also, cardioembolic infarcts are likely to cause large lesions involving the cortex, and thus an association with seizures seems reasonable. Whereas cardioembolic stroke etiology was shown to increase the risk of seizures in some studies,22, 39, 40 others did not find a correlation.15, 17
3.2.2.6 Differentiation of Acute Seizures from Stroke
Seizures are a common mimic of stroke and can complicate rapid decision making at the emergency department.41 Several imaging techniques were devised to enable a differentiation between postictal deficits and stroke. Perfusion CT commonly shows an ictal hyperperfusion, whereas stroke is associated with reduced regional blood flow.42 This pattern enables the differentiation between ongoing seizures or status epilepticus and stroke. However, shortly after termination of seizures the perfusion is rapidly reduced and a regional hypoperfusion can be observed.43 Therefore, perfusion CT might be unreliable to distinguish between postictal deficits and stroke.
Certain MRI sequences might prove more valuable than perfusion CT in the differentiation of late seizures from stroke recurrence. Diffusion-weighted imaging (DWI) predominantly shows increased signal intensity after late-onset seizures around the previously infarcted cortex.44 On arterial spin labeling (ASL), hyperperfusion can be observed after seizures,45 whereas hypoperfusion is seen in acute stroke patients.46 In a small study, changes on ASL were more sensitive for seizures than abnormalities found on DWI.45 Although these results need to be confirmed in larger studies, the high sensitivity of ASL and its easy implementation without the need of a contrast agent might make this technique a valuable tool in differentiating poststroke seizures from acute stroke recurrence in the emergency room.
3.3 Traumatic Brain Injury
3.3.1 Epidemiology
Traumatic brain injury (TBI) accounts for 3% to 7% of all epilepsies1, 47, 48 (Figure 3.1). Similarly, only 2% to 3% of patients presenting with their first unexplained seizure showed traumatic injuries on brain scans.49 These numbers may seem low at first sight, however, TBI is an important cause of epilepsy from a clinical perspective. Seizures are a common problem in TBI patients and for no other cause of epilepsy are the risk factors so well described and easily predictable.50
The relative risk of seizures after severe TBI is markedly elevated by a factor of >90 during the first year and of 17 during the subsequent five years, compared to the general population.51 Especially vulnerable are veterans with penetrating war injuries, causing a relative risk of 580 during the first year and 25 during the subsequent 10 years.52 The risk of seizures clearly correlates with the severity of the injury. The standardized incidence ratios of moderate (7 during the first year and 3 during the subsequent five years) and mild (3 during the first year and 2 during the subsequent five years) TBI are distinctly lower.51 Even for mild injuries the elevated risk of epilepsy persists for at least 10 years.53
Interestingly, nonconvulsive seizures after TBI are common in the intensive care unit and they can be easily missed due to the bad general condition and impaired consciousness of patients. Albeit, if continuous EEG monitoring is used, seizures can be detected in approximately a quarter of monitored patients.54
3.3.2 Imaging Biomarkers
3.3.2.1 Type of Injury
One of the first reports on the value of early imaging in posttraumatic epilepsy showed that focal parenchymal damage or intracerebral hemorrhage on CT scans was associated with posttraumatic seizures.55 This and subsequent studies found that focal lesions on initial CT have a prognostic role for epilepsy and seizures after TBI.51, 52, 56–59 These authors also recognized, that the type of injury markedly influences the likelihood of seizures.
The best evidence exists for the presence of intracerebral hemorrhage and the accumulation of iron in hemosiderin-complexes.51, 52, 56–62 Cortical application of iron is known to elicit seizures and has been used in animal models of posttraumatic epilepsy.63 Hemosiderin can be easily spotted on MRI images with gradient echo (GRE) T2* or susceptibility-weighted imaging (SWI) sequences. One would expect that signs of hemosiderin deposits on brain imaging were associated with poststroke epilepsy, however this seems to be only part of the story. Several investigations showed that the mere presence of hemosiderin does not increase the odds of seizures. However, there was a significant association with hemosiderin deposits surrounded by a hyperintense gliotic wall.61, 62, 64 This observation suggests that not hemosiderin itself but the formation of a gliotic scar after hemorrhage promotes epileptogenesis.
Other well described biomarkers of posttraumatic epilepsy are lesions on CT scans requiring surgical treatment56, 58–61 and depressed skull fractures.51, 57, 58, 65 Some authors also mentioned hydrocephalus60 and open skull injury with brain prolapse as relevant factors.65
The use of specialized MRI sequences might further localize focal changes prone to generating seizures. Magnetization transfer (MT) MR Imaging might be more sensitive to axonal damage than T2 imaging. In a study of MT MRI in TBI patients, the authors demonstrated that patients with MT abnormalities exceeding those seen on T2 imaging were of increased risk of epilepsy after TBI.61 One might speculate, whether this “mismatch” represents particularly vulnerable tissue capable of generating seizures.
3.3.2.2 Hippocampal Sclerosis
A complex association exists between posttraumatic epilepsy and changes of the temporal lobe. Patients with TBI in childhood or young adulthood are at higher risk to develop temporal lobe epilepsy including mesial temporal lobe sclerosis.66 In addition, early posttraumatic seizures can cause structural damage to the temporal lobes. Acute nonconvulsive seizures after TBI were associated with ipsilateral hippocampal sclerosis on follow-up MRI imaging after 6 months.54 Also, posttraumatic epilepsy correlated with the degree of hypoperfusion in the temporal lobes on SPECT one year after the trauma.60 These results suggest that structural changes in the temporal lobes, i.e., hippocampal sclerosis, after brain injury might lead to posttraumatic temporal lobe epilepsy that would, in turn, generate further seizures.
3.3.2.3 Blood-Brain Barrier Dysfunction
Increased scientific interest has recently focused on the role of blood-brain barrier (BBB) disruption in several neurological disorders, including epilepsy and TBI.67 Animal experiments showed that opening the BBB leads to network changes, long-lasting epileptiform activity and eventual neurodegeneration.68, 69 In humans, an MRI based imaging method was devised to semiquantitatively analyze BBB function in vivo.70 Clinical studies show that altered BBB permeability is commonly observed in neurological patients, including those with TBI.71
Based on these findings in TBI and epilepsy patients, two studies analyzed BBB disturbances in posttraumatic epilepsy.72, 73 Both showed a significantly increased BBB permeability in patients with seizures after TBI. The size of BBB dysfunction was also associated with posttraumatic epilepsy and the location of BBB disturbances correlated with focal EEG changes (Figure 3.4). These findings support the notion that lasting BBB disruption may be causally related to the emergence of cortical dysfunction. It is less likely that BBB opening was a consequence of seizures, as increased BBB permeability was observed in patients despite apparent complete medical control of seizures, and no seizures were reported at least 24 hours before the brain scans.
Figure 3.4. Relationship of blood-brain barrier (BBB) dysfunction and seizures after traumatic brain injury (TBI). (A) Statistically significant enhancement of T1 MRI scan in the region surrounding the cortical lesion in patient 21, 10 days following the trauma. (B) Among patients with posttraumatic epilepsy (PTE), the volume of BBB disruption was significantly larger than that of nonepileptic patients. (C) A 34-year-old patient with PTE one month following mild TBI. Power spectrum showing a marked increase in power at 1.125 Hz, taken from an electroencephalography recording, demonstrating abnormal slowing maximal at right frontal and temporal electrodes (inset). (D) sLORETA localizing the pathological signal to the anterior parts of the right middle temporal gyrus (Brodmann area 21, left), and MRI signal enhancement indicating increased BBB permeability localized to the same region (right). *p < .05.
3.4 Brain Tumors
3.4.1 Epidemiology
More than 30% of patients with brain tumors will suffer from seizures during the course of their illness47 (Figure 3.2). Seizures are the presenting symptom of brain tumors in 20% to 40% of patients.74 This makes brain tumors a cerebral disease with the highest likelihood to develop acquired epilepsy, surpassing TBI or cerebrovascular disease.75 Seizures are likely to aggravate neuropsychological problems of tumor patients. Lack of complete seizure control reduces health-related quality of life whereas the use of antiepileptic drugs leads to significant cognitive reductions.76
3.4.2 Imaging Biomarkers
The role of imaging in tumor-associated epilepsy involves the definition of tumor type, tumor location, and changes in peritumoral environment.
3.4.2.1 Tumor Type
There is a clear inverse relationship between the tumor growth rate and the incidence of epilepsy.77, 78 Thus, the primary role of imaging involves visually grading the tumor before histopathological diagnosis can be done. Epileptogenesis is a slow process, requiring months to years to establish focal network changes in the development of epilepsy.78 Low-grade slow-growing tumors have the highest seizure frequency. These include dysembryoblastic neuroepithelial tumors (up to 100%), oligodendroglioma (60–85%), low-grade astrocytomas (60–85%), and anaplastic astrocytoma (45–70%).74, 78–81 MRI features of these tumors include a homogenous structure with no or minimal contrast enhancement. Oligodendroglial tumors characteristically show calcifications, best spotted on CT or susceptibility-weighted MR images.
Nevertheless, a nonnegligible proportion of patients with faster growing tumors like glioblastoma multiforme (15–30%) or cerebral metastasis (20–35%) are also likely to develop seizures.74, 75, 77–79, 81 These tumors are thought to induce epilepsy through abrupt tissue damage due to necrosis or deposition of hemosiderin caused by their rapid growth.78 Glioblastoma multiforme typically appears on MRI as a T2 hyperintense mass with irregular contrast enhancement and vasogenic edema. MR features of cerebral metastases involve single or multiple nodular T2 hyperintense structures surrounded with vasogenic edema and, commonly, annular or uniform contrast enhancement.
3.4.2.2 Tumor Location, Size, and Hemosiderin Deposits
Location of the brain tumor significantly influences the incidence of seizures.80 Tumors located in the frontal, temporal, and parietal lobes are most likely to be associated with epilepsy, whereas occipital or infratentorial tumors have a low propensity for seizures.74, 80 Seizures also occur infrequently, if only white matter structures are affected.77
Other factors that influence the occurrence of seizures are the size of the tumor and the presence of hemorrhage. Tumor size and the number of lesions are positively associated with epilepsy.82–84 The presence of hemosiderin deposits on susceptibility-weighted MR images (SWI) predicted seizures in patients with brain metastasis and glioblastoma. An even stronger association was seen if SWI lesions involved the cerebral cortex.84
3.4.2.3 Tuberous Sclerosis Complex
Tuberous sclerosis complex (TSC) is an autosomal dominant disorder with a high spontaneous mutation rate, leading to tumorous growth in multiple organs including the brain. More than 80% of children with TSC have epilepsy due to multiple brain tubers and some can be treated surgically. To ensure the success of surgery, the clinician needs to determine which of these tubers are epileptogenic and will need to be resected.
A functional imaging method was devised to noninvasively localize the epileptogenic tubers. PET with the α-[11C]methyl-L-tryptophan ([11C]AMT) tracer showed decreased binding in those tubers, which were not expected to generate seizures. Conversely, epileptogenic tubers had an equal or increased uptake ratio compared to the normal cortex85 (Figure 3.5). For tubers with an uptake ratio of ≥1 compared to normal tissue, [11C]AMT-PET shows a specificity of 80–90% for the detection of epileptogenicity.86, 87 Although this concept has also been utilized in children with intractable epilepsy, it has not yet been successfully adapted to patients with seizures due to other types of brain tumors.88
Figure 3.5. Comparison of MRI with fluorodeoxyglucose (FDG) PET and [11C]AMT-PET in an 8-year-old girl with tuberous sclerosis complex and intractable epilepsy. (A) MRI scan showing large tuber in the right central/parietal region (bold arrow) as well as other small lesions (thin arrows). (B) The FDG PET shows cortical hypometabolism in the same regions as the lesions on the MRI (arrows). (C) The [11C]AMT-PET displays decreased tracer uptake in the location of small tubers (thin arrow). The large right central tuber (bold arrow) shows a 110% increase of [11C]AMT uptake.
Another noninvasive method to identify epileptogenic areas in TSC is EEG-fMRI. A small study in five children found interictal activations in and around epileptogenic tubers.89 These activations were more widespread compared to the abnormalities found on PET or SPECT studies, suggesting that a multifocal network generates interictal discharges in TSC. Although this method might be more sensitive for hyperexcitable networks distant to the lesions, it might be less specific for the evaluation of critical tubers that should be surgically resected.
3.5 Other Diseases
3.5.1 Multiple Sclerosis
Traditionally, multiple sclerosis (MS) was regarded as a disease selectively affecting the white matter. As such, a subcortical disorder would be unlikely to have a relevant propensity for epileptic seizures. However, the increased frequency of epilepsy in MS has been well documented within the last decades. Population-based studies show a prevalence of around 3% (Figure 3.2), corresponding to a relative risk of 3 to 4 compared to the general population.90, 91 Occurrence of seizures is associated with early MS onset and increased disease severity.92, 93
However, as evidence for cortical involvement in MS started to accumulate during the last years, there was a major paradigm shift.94, 95 Nowadays, there is no doubt that MS also affects cortical structures. Although older findings inconsistently showed an association of seizures with corticosubcortical lesions,96–101 these studies have likely underestimated the prevalence of isolated cortical lesions. Conventional MR sequences used in these studies, including fluid-attenuated inversion recovery (FLAIR) and T2-weighted spin echo (T2SE), cannot detect isolated intracortical inflammation, as was demonstrated with histopathological evidence.
The advent of modern double inversion recovery (DIR) sequences markedly improved the detection of cortical plaques (Figure 3.6). Studies performed with this technique consistently find a correlation of intracortical inflammation with seizures. Intracortical lesions were observed in 90% of MS patients with seizures, compared to 48% of those without (p = .001).102 MS patients with epilepsy showed five times more intracortical lesions, and their volume was six times larger. A “worm-like” shape particularly correlated with seizures. On the other hand, no significant differences were observed with regard to the number and volume of juxtacortical lesions and T2 white-matter lesion volume.
Figure 3.6. Four types of cortical lesion identified in multiple sclerosis patients by double inversion recovery (DIR) imaging. (A) Mixed white matter and gray matter lesion (type I). (B) Intracortical lesions (type II). (C) Subpial lesion (type III). (D) “Worm-like” lesion (type IV).
These results underline the importance of cortical inflammation for epileptogenesis in MS. Additionally, MS patients with seizures have a more rapidly and severely evolving cortical pathology. During a 3-year study, these patients had a higher accumulation of intracortical lesions and a faster reduction of gray matter fraction compared to those without seizures.103 Conversely, the two groups did not differ in the number of new white matter lesions. Further evidence comes from a histopathological study that found cortical plaques and atrophy of the temporal lobe to be associated with seizures in MS.104
3.5.2 Alzheimer’s Disease
Seizures as a comorbidity of neurodegenerative disorders are particularly well described for Alzheimer’s disease (AD). Patients with Alzheimer’s dementia are at sevenfold risk of seizures compared to age-matched controls105 and account for around 2% of all epilepsy.106 Due to the high prevalence of AD in the aging population, these numbers are of increasing relevance in elderly patients.
Several clinical variables have been associated with seizures in AD. The most robust correlation is described for young age at dementia onset.107–109 This is particularly apparent in genetic forms of dementia as many human AD gene mutations leading to increased β-amyloid also cause epilepsy.110 Other clinical variables correlating with seizures were antipsychotic drug use, African American race, epileptic discharges on EEG, and greater cognitive impairment at baseline.107, 111
Imaging predictors of epilepsy in AD have not been described yet. One short report found hippocampal atrophy in all AD patients with seizures.112 However, this could also be attributed to increased disease severity in these patients. This significant gap of knowledge will need to be addressed by future studies. These will concentrate on the complex bidirectional interplay between dementia and epilepsy. On one hand AD can cause seizures; on the other hand epilepsy patients are likely to develop progressive cognitive deficits and, ultimately, dementia. Future neuroimaging studies will focus on the common pathomechanisms of these disorders including hyperexcitable temporal networks, amyloid and tau deposition, BBB disruption, and changes of NMDA receptor transmission.110, 113, 114
References





















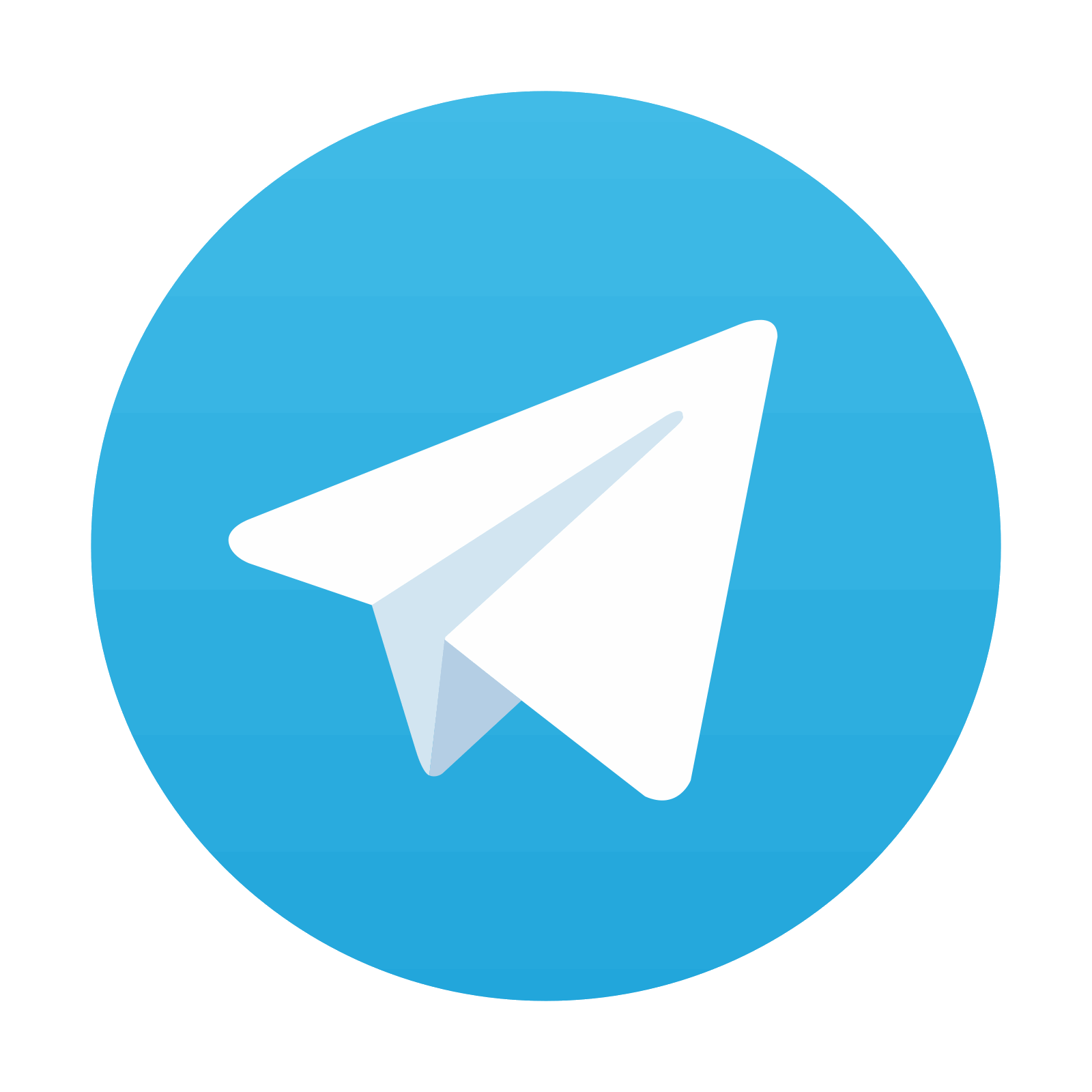
Stay updated, free articles. Join our Telegram channel
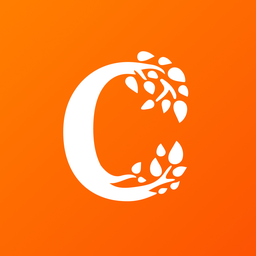
Full access? Get Clinical Tree
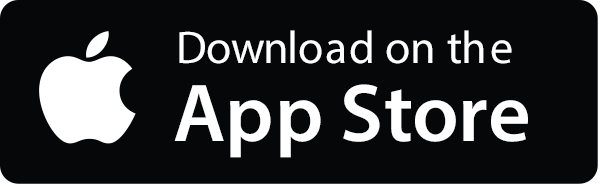
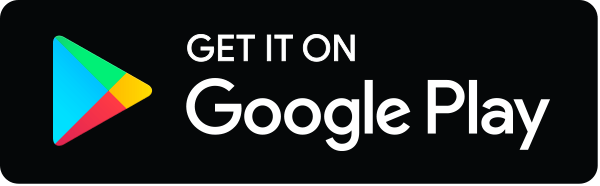
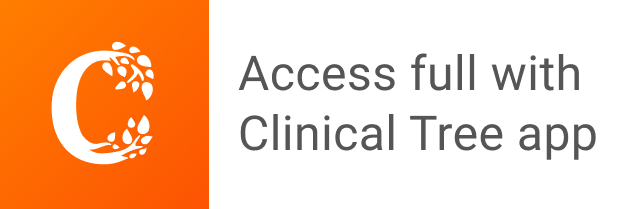