High-Frequency EEG Activity
Jean Gotman
Nathan E. Crone
INTRODUCTION
The electroencephalogram (EEG) is traditionally recorded and clinically interpreted with a low-pass filter set at 60 or 70 Hz. The most common EEG activities are divided into the delta, theta, alpha, and beta bands, the latter term usually used for activity extending up to ˜30 Hz. There has been much interest recently in the gamma band, a term commonly used for activity faster than beta (>30 Hz). Because of considerable variability in the frequency bounds of gamma band activity reported across different studies, it may be premature, or even altogether inappropriate to select a specific frequency range to define it. Nevertheless, there is a practical need for terminological consistency in order to facilitate communication in both scientific discourse and translation to clinical practice. Based on accumulating evidence from studies of both physiologic and pathologic responses in gamma frequencies (see below), it may be useful to arbitrarily, but provisionally define the gamma band as 30 to 70 Hz. It has recently been discovered that frequencies higher than 70 Hz can also be recorded and can have both physiologic and pathologic significance. A variety of terms have been used to describe physiologic EEG activity above 70 Hz, for example, “high-gamma” (1,2) and the chi-band (3). In the context of epilepsy, pathologic patterns at high frequencies have also been described, for example: ripples between 100 and 200 Hz (sometimes between 80 and 160 Hz, sometimes between 100 and 250 Hz), fast ripples between 160, 200, or 250 Hz and 500 or 600 Hz. These events have other properties besides their frequency, and in general, we think that frequency bands should be defined independently from specific EEG phenomena at a time when much exploration is taking place. Rather than using a name, we will therefore refer to bands by their boundaries (e.g., the 100- to 250-Hz band).
The Skull, the Generator Volume, and the Electrodes
It is often said and sometimes written that “the skull filters out high frequencies.” This would imply that high frequencies, for instance above 60 Hz, would be more attenuated by the skull than lower frequencies, making it essentially impossible to record high frequencies except with intracranial electrodes or with magnetoencephalography (MEG) that is not sensitive to skull conductivity. In fact the skull does not attenuate any frequency until approximately 10,000 Hz. This has been clearly demonstrated in the excellent study of Oostendorp et al. (4). There seems to be, however, an inverse relationship between the frequency of an EEG rhythm and the volume of brain that generates it. For instance, very large regions can generate synchronous delta activity, and smaller regions can generate synchronous higher frequencies. As very high frequencies are generated in a very small volume, they are unlikely to be “seen” from the scalp, simply because of the small angle under which they would be seen (5). For the same reason (small generator volume), high frequencies usually have low amplitude and the skull attenuation reduces this amplitude further. So it is indeed unlikely that high frequencies can be seen from the scalp, but it is not because they are filtered by the skull; it is because of the characteristics of their generator. For these reasons (distance from generator to sensor and small amplitude of signal), it may be just as difficult to record high frequencies with MEG as it is with scalp EEG.
In the context of a small generator volume, the size of recording electrodes becomes a potentially important factor, although the question of electrode size has rarely been addressed in the field of intracranial EEG (iEEG). If a generator is on the order of a few hundred microns, two issues can be raised. The first is that of spatial sampling: if such small generators are scattered and present in a small number of locations, the likelihood that an electrode would be in their vicinity is low. This is one extreme of the general problem of poor spatial sampling of intracranial investigations. The second one is that an electrode larger than the field generator and in contact with it could significantly alter that field, and an electrode much larger than the field generator could attenuate it, possibly to the point of making it undetectable. It is very difficult to know the size of a generator, unless one performs a systematic exploration with microelectrodes (6). It is therefore not easy to determine the optimal electrode size. Electrodes have usually been designed to allow electrical stimulation without an excessive current density (7,8); for this purpose, a relatively large contact surface is important but this requirement may be in conflict with that of optimizing the recording of high-frequency activity.
It may also be useful to discuss some terminology issues. In the animal experimentation domain, the notion of “local field potential” is often used. For instance filtering an extracellular microelectrode recording such that only activity below 100 Hz, or below 500 Hz, remains (i.e., removing action potentials), is said to produce the local field potential (LFP). This extracellular potential fluctuation is not easily differentiated from the EEG, as recorded locally with a small electrode. Although the term “EEG” is most often associated with relatively large electrodes, there is nothing in the definition of the EEG that implies electrode size. It is rather defined as potential fluctuations within a certain frequency range. From our point of view, there is no formal difference between LFP and EEG, but the terms tend to be used in different contexts.
We will first discuss the high frequencies that are recorded in the context of normal function. These have been documented in experimental animals as well as in humans in response to specific sensory, motor, or cognitive activities. In humans, some of these high frequencies can be recorded from the scalp but most were investigated in patients with intracerebral electrodes in the context of the evaluation of the surgical treatment of their medically refractory epilepsy. We will then discuss the high-frequency activities that have been related to focal epilepsy, here again investigated in experimental animals and in patients with intracerebral electrodes.
HIGH-FREQUENCY ACTIVITY AND NORMAL CORTICAL FUNCTION
Experimental Background and Significance
The functional relevance of high-frequency EEG activity in normal brain physiology has been the subject of many investigations over the past several decades in both animals and humans and is still hotly debated. Some of the earliest studies using EEG recognized that cortical activation was associated with the disappearance of low-frequency activity (alpha) and the appearance of fast activity, though the extent of this fast activity was difficult to ascertain given the technical limitations of existing equipment (9, 10 and 11). Subsequent microelectrode recordings in rabbit olfactory cortex (12) showed that different odors elicited different spatial patterns in the amplitude of sniff-triggered gamma oscillations (38 to 80 Hz), suggesting a mechanistic role in stimulus discrimination. Similar studies in cat and rat revealed oscillations with a similar frequency range (13). A decade later, local field recordings in cat visual cortex found that oscillatory neuronal firing in a frequency range of 40 to 60 Hz can become synchronized during visual stimulation between spatially separate columns (14), between areas 17 and 18 (15), and even between areas 17 of the two hemispheres (16), and that this synchronization depends on global stimulus properties. Similar activity, ranging up to 35 and 50 Hz was recorded in monkey sensorimotor cortex during voluntary hand movements (17,18). In these studies bursts of single unit activity were commonly synchronized with LFP oscillations, suggesting that these oscillations facilitated and/or were facilitated by the synchronization of neuronal firing between spatially segregated but functionally related neurons.
Synchronization of neural firing has been hypothesized to form the basis for temporal coding by which temporary assemblies of neurons represent higher-order, or global, stimulus properties (19). This mechanism of temporal coding (20) was recognized as an exciting potential solution to the binding problem associated with psychophysiologic phenomena such as sensory segmentation and invariant object recognition. More recent theoretical refinements have further proposed that subthreshold gamma oscillations, driven by synchronous inhibitory neuron firing, produce temporal windows for cortical-cortical communication, whereby coherently oscillating ensembles of neurons interact more effectively because their communication windows are open at the same time (21). Another theory proposes that rhythmic gamma oscillations from inhibitory interneuronal firing interact with excitatory inputs to pyramidal cells such that more excited cells fire earlier in the gamma cycle. This recording of the amplitude of excitatory drive into phase values relative to the gamma cycle would provide a more efficient coding mechanism by enabling the readout of amplitude information within a single gamma cycle without requiring the temporal integration of firing rates (22).
Band-Limited Gamma Oscillations
A common feature of the aforementioned theories relating high-frequency EEG (gamma) activity to neural coding and cortical function is that they all more or less require rhythmic oscillations of membrane potentials and/or action potentials at relatively stable, that is, band-limited, gamma frequencies. Although synchronous neural activity is not necessarily oscillatory and temporal coding could conceivably exist without oscillations, the explanatory potential of band-limited gamma oscillations has been compelling (23). Perhaps inspired by this, many investigators have attempted to correlate band-limited gamma oscillations in human EEG with cognitive and perceptual operations in cortex.
Early attempts to relate band-limited gamma oscillations to human cortical function were designed to demonstrate taskspecific lateralization of 40-Hz activity in scalp-recorded EEG during long blocks of task performance. Although compensations had to be made for 40-Hz EMG artifact, these studies did show lateralization of 40 Hz to the left hemisphere during verbal tasks (24), and to contralateral central scalp region during both simple and complex choice reaction time tasks (25,26). However, like block designs in fMRI experiments, estimates of gamma activity in long temporal segments during both resting and activated conditions can be contaminated with extraneous behavioral fluctuations. Furthermore, this block-design approach does not exploit the fine temporal scale at which gamma activity can change during task performance. For these reasons, event-related designs have been used much more extensively when studying gamma responses.
Phase-Locked Gamma Responses
Using event-related analyses of MEG, Pantev et al. (27) observed band-limited gamma responses to tone bursts at 35 to 40 Hz. This was consistent with previous reports of 40-Hz clicks driving large steady-state evoked responses in auditory cortex (28). Notably, the gamma-band responses (GBRs) observed by Pantev et al. (27) were obtained by filtering event-related potentials (ERPs) produced by averaging raw signal from many individual trials in the time domain. This procedure reveals phase-locked evoked GBR, consisting of gamma components in the ERP. It is important to distinguish these evoked GBRs from induced gamma band activity that is time-locked, but not phase-locked (29) (Fig. 37.1; see the section “Non-Phase-Locked Gamma Responses”).
Phase-locked gamma responses have been investigated in a variety of experimental contexts. In many cases narrow band-pass filters have been used to focus on ERP components in and around 40 Hz. This practice is potentially susceptible to artifacts from filtering ERP impulses containing a wide range of
frequencies. Nevertheless, evoked GBRs have been observed in both animals and humans during cortical activation in a variety of functional-anatomic domains (30, 31 and 32), and in humans this class of responses has been used to explore mind-brain relationships (33,34).
frequencies. Nevertheless, evoked GBRs have been observed in both animals and humans during cortical activation in a variety of functional-anatomic domains (30, 31 and 32), and in humans this class of responses has been used to explore mind-brain relationships (33,34).
![]() Figure 37.1 Signal analysis of phase-locked and non-phase-locked responses using band-pass filtering. Schematic of signal analyses for one channel of intracranial EEG recorded over dominant (left) superior temporal gyrus (filled circle on brain) during an auditory speech discrimination task. To minimize the contribution of phase-locked responses to subsequent analyses of non-phase-locked responses, the ERP may be subtracted from the raw EEG signal in each individual trial (depicted in steps A, B, and C; for alternative approaches, see Kalcher et al. (42) and Trautner et al. (69)). In this approach, the raw signal (A) is averaged across N trials to obtain the event-related potential (ERP, B), and the ERP is then subtracted from each individual trial (C) prior to band-pass filtering (D, 80 to 100 Hz in this illustration). Band-pass filtered signals from each individual trial are then squared to obtain power values (E). These band-specific power estimates, which are all positive, may then be averaged across trials to obtain the power average (F), or submitted to statistical analyses to calculate the % change in poststimulus power from prestimulus baseline power (G). Event-related power increases (F,G) are also known as induced responses and are dominated by non-phase-locked response components. Note the variability in the latency and magnitude of these responses across individual trials (E). (From Crone NE, Boatman D, Gordon B, et al. Induced electrocorticographic gamma activity during auditory perception. Clin Neurophysiol. 2001;112(4):565-582.) |
In addition to ERP components in the gamma band, ERP components have also been observed in even higher frequencies, for example, ultrafast frequencies (400 to 1000 Hz), in animals and humans. For example, both EEG and MEG recordings (Fig. 37.2) have shown that somatosensory evoked potentials from electrical stimulation of the median nerve contain a brief (10 to 15 msec) burst of ˜600-Hz spikelike wavelets, called sigma bursts (35, 36 and 37). Sigma bursts temporally overlap the thalamic P15 component and the N20 primary cortical response and, based on converging evidence from animals and humans, are thought to represent both far-field and near-field potentials generated by highly synchronized population spikes in cuneothalamic and thalamocortical relay cells and a variety of cortical neurons. These sigma bursts are diminished during sleep, particularly slow-wave sleep, though the N20 is not (38). EEG wavelet bursts at similar frequencies have been observed in animals (39). In awake monkeys (40), for example, a subset of SI units exhibits bursts of spikes and single spikes that are phase-locked to surface EEG wavelets elicited by electrical median nerve stimuli (Fig. 37.3). Because many central synapses transmit bursts but filter out single spikes, coincident spike bursts from thalamocortical relay neurons may drive postsynaptic cortical cells more efficiently than single spikes. Furthermore, they may also be capable of richer coding because intraburst spike frequency can convey graded information about sensory stimuli while bursts of different durations can code different stimulus features.
Non-Phase-Locked Gamma Responses
Averaging electrophysiologic (EEG, MEG, LFP, etc.) responses in the time domain extracts phase-locked signal components as ERPs and discards non-phase-locked components as noise. In contrast, averaging these responses in the frequency domain focuses on event-related changes in the spectral energy of responses that may have both phase-locked and non-phase-locked components (41). The latter approach is still temporally linked to an event across multiple trials, but the result is not limited to phase-locked components, as in ERPs. Different methods have been used to recognize and minimize the contribution of phase-locked components (ERPs) to analyses of non-phase-locked electrophysiologic responses (42,43).
However, the distinction between phase-locked responses (ERPs) and non-phase-locked increases in signal energy (often termed “induced” responses) may depend more on the methods by which they are extracted, than on fundamental differences between their generators.
However, the distinction between phase-locked responses (ERPs) and non-phase-locked increases in signal energy (often termed “induced” responses) may depend more on the methods by which they are extracted, than on fundamental differences between their generators.
Averaging in the time domain necessarily yields phase-locked responses. However, significant variability (jitter) in the latency (or phase) of ERPs can distort their appearance in time-averaged responses. High-frequency components of electrophysiologic responses are more susceptible to latency jitter, and there is usually more jitter of these responses (and their corresponding cognitive processes) at longer latencies. This probably explains why ERPs with high-frequency components (e.g., the sigma bursts described above) are usually confined to early latencies. On the other hand, ERPs at longer latencies (e.g., P300) usually consist of low-frequency components that are more resistant to jitter (44,45). Because averaging in the frequency domain does not require phase-locking, it may be better suited to investigate cortical processing at longer or more variable latencies and to investigate high-frequency electrophysiologic responses at longer latencies. It may therefore be useful to conceive of electrophysiologic responses as having different combinations of frequencies, latencies, and phase-locking. Nevertheless, the distinction between phase-locked and non-phase-locked responses is often still a practical one. Furthermore, many studies suggest that these different classes of EEG responses may have distinct functional response properties (29,46,47).
Although early studies of gamma activity using block designs, strictly speaking, did relate non-phase-locked gamma activity to human cortical function, one of the first event-related analyses of this activity, that is, on a temporal scale commensurate with task performance, was made by Pfurtscheller et al. (48), who demonstrated a lateralized increase in 40-Hz activity during self-paced finger movements in three subjects. One of these subjects was later shown to have a somatotopic pattern of this activity during movements of the tongue, fingers, and toes (49). Similar non-phase-locked gamma activity has also been observed in a variety of experimental contexts, for example, during the perception of illusory triangles (50).
Broadband “High Gamma” Activity
As mentioned above, most noninvasive studies of gamma activity vis-à-vis normal cortical function, until recently, have focused on relatively narrow band-limited gamma frequencies in and around 40 Hz. These studies were motivated in part by previous animal studies of band-limited gamma oscillations and by the aforementioned theories of cortical coding that necessitate rhythmic oscillatory activity at consistent, narrowband gamma frequencies. iEEG recordings in patients undergoing epilepsy surgery, however, have allowed the investigation of physiologic gamma responses across a broader range of frequencies than those studied in humans by noninvasive methods. These studies have revealed event-related, non-phase-locked responses in gamma frequencies higher than those previously studied noninvasively (2). In addition, these “high gamma” responses have had a characteristically broadband spectral profile that spans a large range of frequencies. The lower and upper frequency boundaries of these responses have been quite variable, but they have most commonly ranged from ˜60 to ˜200 Hz, with the majority of event-related energy changes occurring between 80 and 150 Hz (51,52).
Across a variety of iEEG studies broadband high gamma responses have exhibited functional response properties that distinguish them from both phase-locked responses (ERPs) and non-phase-locked responses previously observed with scalp EEG in other frequency bands (2). For example, the temporal and spatial distributions of these responses are typically more discrete and/or functionally specific for task-related cortical activation than other electrophysiologic responses. Furthermore, broadband high gamma responses have been demonstrated in a great variety of functional-anatomic domains, including motor cortex (1,53, 54, 55, 56, 57, 58, 59, 60 and 61), frontal eye fields (62), somatosensory cortex (63, 64, 65 and 66), auditory cortex (43,67, 68 and 69), visual cortex (70, 71, 72, 73 and 74), olfactory cortex (75), and language cortex (74,76, 77, 78 and 79). The functional-anatomic ubiquity of these responses suggests that they may serve as a general electrophysiologic index of cortical processing.
Motor Cortex
Investigations of broadband high gamma responses in motor cortex have provided many insights into their functional response properties. In a study using a visually cued motor task (1,80) power augmentation was observed from 75 to 100 Hz within somatotopically defined regions of sensorimotor cortex contralateral to the cued movement (1) and corresponded well to electrocortical stimulation maps of motor function. The temporal patterns of these responses were also quite specific, occurring in brief bursts limited to the onset and offset (81) of movement, with latencies that covaried with the latencies of movement onset/offset. In another study using self-paced finger and wrist movements, Ohara et al. (53) observed non-phase-locked gamma activity in S1 and M1 extending up to 90 Hz. High gamma activity (60 to 90 Hz) in particular was time-locked to movement onset and was short-lived after it. Pfurtscheller et al. (54) also observed broadband high gamma activity (60 to 90 Hz) over sensorimotor cortex while subjects performed self-paced tongue and finger movements. As in the previous studies, the topographic pattern of high gamma activity was more discrete and somatotopically specific than the more widespread power changes in mu (alpha) and beta bands, and its temporal pattern was also briefer, corresponding to movement onset.
In the largest series to date (22 subjects) of iEEG recordings in sensorimotor cortex, Miller et al. (56) compared the topographic patterns of power suppression in low frequencies (8 to 32 Hz) versus power augmentation in high gamma frequencies (76 to 100 Hz) during a variety of motor tasks. As in the previously mentioned studies, high gamma responses had a more focused spatial distribution than did power suppression in low frequencies. In addition, these responses had a somatotopic organization corresponding to the movement of different body parts and corresponded well to the results of electrocortical stimulation mapping. This and other reports (57,82,83) suggest that high-frequency responses may be a useful tool for mapping motor function in patients undergoing surgical resections near or within motor cortex.
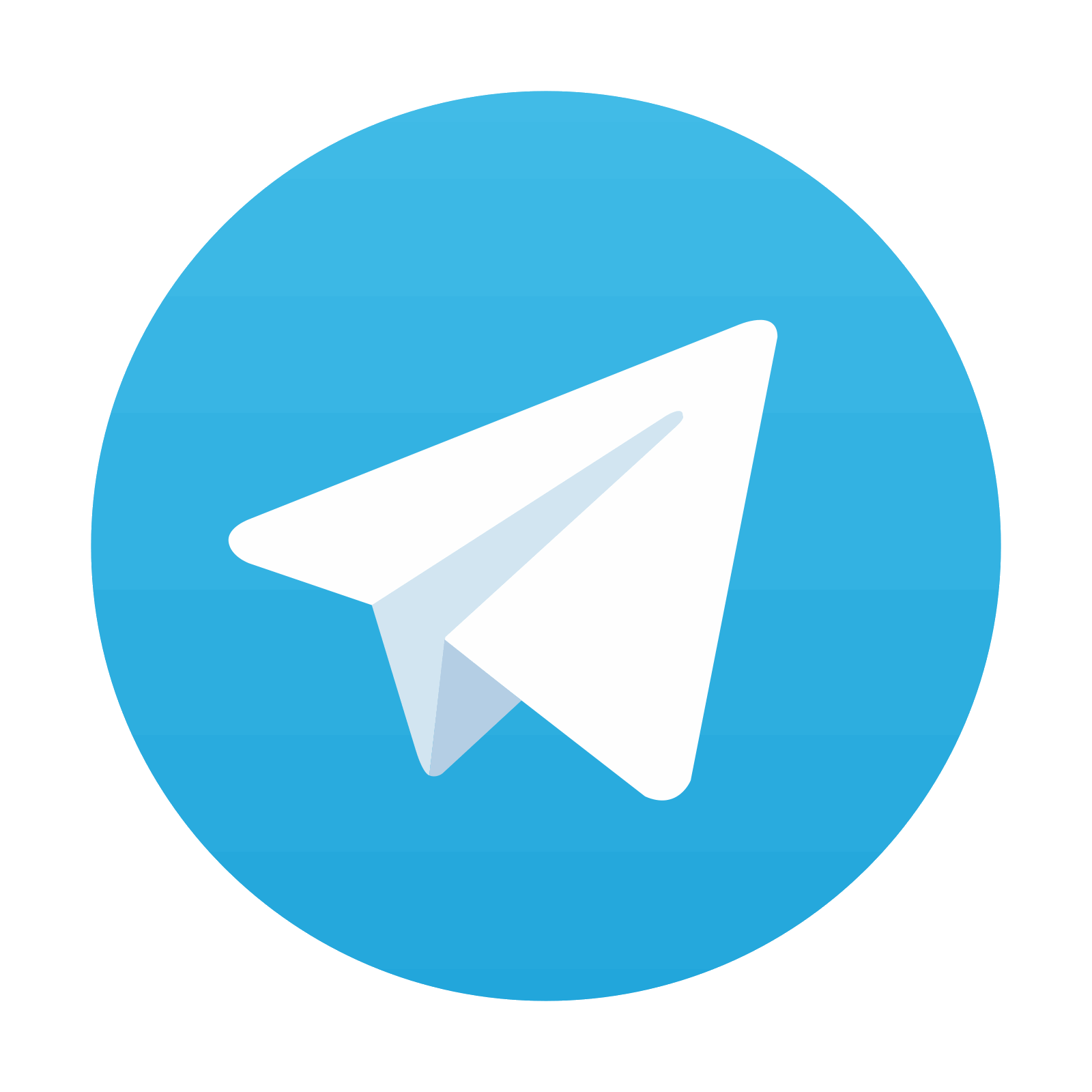
Stay updated, free articles. Join our Telegram channel
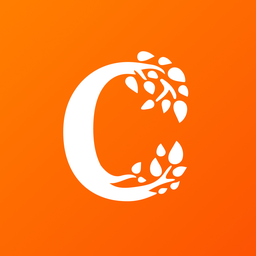
Full access? Get Clinical Tree
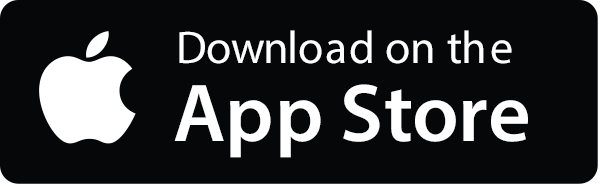
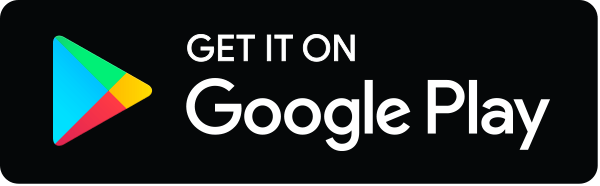