CHAPTER 253 Interstitial and Intracavitary Irradiation of Brain Tumors
Brachytherapy is the practice of administering therapeutic radiation by means of a source placed a short distance from or within the body part being treated, as opposed to teletherapy, in which the radiation source is outside the body, several feet away. Brachytherapy attempts to improve the therapeutic ratio by allowing the delivery of high doses of radiation to localized tumor volumes or resection margins without significant irradiation of surrounding normal brain. The first examples of brain tumor brachytherapy used radium as the radiation source. In 1912, Hirsch inserted a radium probe into the sella of an acromegalic woman.1 Two years later, Frazier implanted radium into a malignant glioma tumor bed at the time of a craniotomy.1 The development of new radioisotopes in the late 1940s and early 1950s, coupled with the development of frame-based stereotactic systems, brought about renewed interest in brain tumor brachytherapy.
Two types of brain tumor brachytherapy quickly emerged: intracavitary, or placement of a radiation source in cystic lesions in which the target is a thin rim of neuroepithelial tissue in the cyst wall, and interstitial, or placement of a radiation source in solid tissue, either inoperable tumors or the margins of resection cavities after subtotal or total tumor resection. Intracavitary brachytherapy was performed initially for craniopharyngioma in 1950 by Leksell, who used a phosphorus 32 (32P) sodium phosphate solution to treat a 40-year-old man with recurrent cystic craniopharyngioma.2 Since then, intracavitary brachytherapy has been used mainly outside North America.
Within North America, there has been extensive experience with interstitial brachytherapy in the form of iodine 125 (125I) implants. These 125I implants began as high-activity temporary implants in the form of stereotactically placed catheters containing small titanium capsules or “seeds” within which reside resin balls containing the radioactive isotope. These catheters had to be removed to prevent toxicity from the radiation, with removal accomplished in a bedside or outpatient procedure involving a small scalp incision followed by catheter removal and suturing of the scalp. Although high-activity temporary implants deliver higher doses in shorter periods, there has been a transition over the past decade toward low-activity permanent implants that deliver radiation over a longer period. Low-activity permanent implants are free individual titanium capsules 4.5 mm long and 0.8 mm in diameter that are placed in the walls of the resection cavity; within the capsules reside the 0.67-mCi 125I radioactive sources (Fig. 253-1). Low-activity permanent implants may be associated with a lower rate of reoperation for radiation necrosis. A report in which low-activity permanent 125I implants were used to treat metastases found that 2 of 26 (8%) patients required reoperation for radiation necrosis3 as opposed to 1 of 6 (17%) in another report using temporary high-activity 125I implants to treat metastases4 (Table 253-1).4–13 Similarly, low-activity permanent 125I implants are associated with a slightly lower rate of reoperation for radiation necrosis than are high-activity temporary 125I implants when used to treat primary and recurrent glioblastoma (Table 253-1).
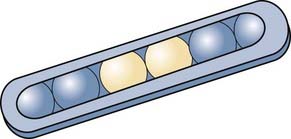
FIGURE 253-1 Illustration of low-activity, permanent iodine 125 seeds, which are close to the size of a grain of rice.
TABLE 253-1 Comparison of Temporary High-Activity and Permanent Low-Activity 125I Seeds with Temporary High-Activity 125I GliaSite Implants
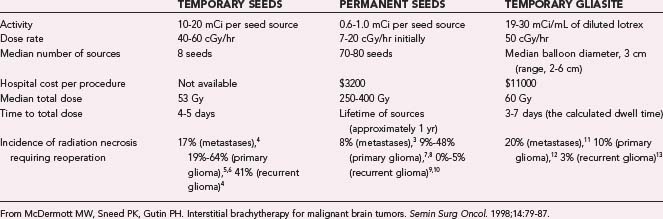
Early studies identified limited homogeneity and reproducibility with low-activity permanent 125I seeds, specifically, (1) inhomogeneous distribution of the radiation dose within a patient’s resection cavity because of spatial asymmetry in the location of the seeds and (2) patient-to-patient heterogeneity, which makes efficacy studies difficult because of an inability to reproduce the spatial distribution of seeds from patient to patient. Seeking to overcome these limitations, Proxima Therapeutics (Alpharetta, GA) returned to high-activity temporary 125I implants when they designed GliaSite, a balloon catheter system that is implanted at the time of tumor craniotomy. The distal end of the balloon lies in the tumor cavity, whereas the proximal injection port lies under the scalp and is filled with 125I-containing solution a few days after the craniotomy. Once the brachytherapy is completed, the patient undergoes an outpatient procedure to remove the implant. Although GliaSite provides better homogeneity within the resection cavity than permanent low-activity 125I seeds do, the dose distribution outside the cavity can be nonconformal with GliaSite because the GliaSite dose distribution is always a sphere whereas the cavity shape varies, which means that targeted positioning of 125I seeds may enable more uniform dose distribution outside the cavity than possible with GliaSite. The clinical significance of the homogeneous intracavitary dose distribution of GliaSite versus the potentially more homogeneous extracavitary dose distribution of 125I seeds remains to be determined. The safety of GliaSite was proved in a study of 21 patients with recurrent glioblastoma published in 2003.14 The radiation necrosis associated with the original catheter-based high-activity 125I implants occurred to a comparable extent when treating metastases with GliaSite but was not as common when treating primary or recurrent glioblastoma with GliaSite (see Table 253-1); however, this observation will require confirmation in larger series. Although 125I low-activity seeds can be applied to tumor of any size and are relatively inexpensive ($3200 is the cost of a typical implant), GliaSite is limited to tumors less than 6 cm in diameter because of limitations in balloon size and can cost $11,000 for a typical implant (see Table 253-1). Since the year 2000, most brachytherapy studies have involved low-activity permanent 125I seeds or high-activity temporary 125I GliaSite implants, with catheters containing high-activity seeds falling out of favor. The biggest alternative to these brachytherapy techniques for brain tumors has been the increased availability and use of radiosurgery. This chapter reviews the radiobiology of brachytherapy and its success in treating various brain tumors, including how it compares with radiosurgery.
Radiobiology
The characteristics of the common isotopes used for brachytherapy are listed in Table 253-2. Intracavitary treatment tends to use low-energy β-emitting sources such as 32P or yttrium 90 (90Y), with which most of the radiation dose is deposited in the first few millimeters, perfect when the source is in the center of a cyst with a thin wall that is the target. Pathologic specimens from patients treated in this manner demonstrate that the epithelial lining cells of the cyst wall are destroyed and replaced largely by fibrous tissue.15 By comparing the activity of cyst fluid aspirated a certain number of days after instillation of the isotope with isotope activity measured on an isotope scintillation scan, Fig and colleagues showed that the larger the cyst, the higher the actual dose to the cyst wall.16
The type of ionizing radiation used, the total activity of the sources, and the dose rate are important determinants of the biologic effect of any radiation dose. In general, the lower the dose rate, the smaller the biologic effect of any given dose, particularly over the range of 0.01 to 1 Gy/min.1,17 Dose rates from interstitial brachytherapy are usually less than 0.6 Gy/hr, much lower than the 1.8 to 2 Gy/min exhibited by conventional linear accelerators. However, there are four distinct advantages of continuous low-dose irradiation. The first therapeutic advantage of continuous low-dose irradiation that occurs with interstitial brachytherapy relates to differences in repair of sublethal radiation-induced damage between tumor and normal tissue, with normal tissue being more efficient in this regard.18 However, the higher the dose rate, the lower the total dose at which normal tissue DNA damage is observed, thereby lessening the difference in repair of radiation-induced DNA damage between tumor and normal tissue.19 A second therapeutic advantage of continuous irradiation is that it allows time for cells in the radiation-resistant G1 phase of the cell cycle to enter the more sensitive G2 and M phases while radiation is still being delivered. A third therapeutic advantage of continuous low-dose irradiation is that hypoxic cells, traditionally regarded as being resistant to conventional radiation, are relatively more sensitive to continuous low-dose irradiation.20 A fourth therapeutic advantage of continuous low-dose irradiation is that despite using a lower dose, the dose of brachytherapy is still sufficient to inhibit mitosis but is present for up to six cell cycles whereas conventional radiotherapy is present for only one cell cycle and uses a dose 2 to 3 times that needed to inhibit mitosis.
Implantation Technique
Interstitial Brachytherapy
High-Activity Implants
Originally, stereotactic catheters were used to place high-activity temporary implants, but this technique has since been replaced by GliaSite implantation. After craniotomy for maximal resection, a 2-, 3-, or 4-cm GliaSite balloon catheter device is placed in the resection cavity, with the neurosurgeon selecting the size closest to but smaller than the diameter of the resection cavity. The GliaSite device is filled with saline and iodinated contrast material, and the catheter access port is secured to the cranium (Fig. 253-2). After closure in the usual method, gadolinium-enhanced magnetic resonance imaging (MRI) is performed for confirmation of catheter placement and dosimetric planning. In the radiation oncology clinic 2 to 6 weeks after surgery, the radiation oncologist removes a volume of iodinated contrast material equal to the planned injection volume of Iotrex solution and then loads the GliaSite balloon with the solution. The dwell time necessary to deliver the prescribed total dose is calculated by integrating the dose rate at the prescription point with the 60.2-day half-life of 125I. Some, but not all institutions admit the patient to the hospital during the dwell time. Patients also receive a regimen of potassium iodide to block any uptake of 125I by the thyroid in the unlikely event of balloon failure. The balloon catheter must be removed by the neurosurgeon in an outpatient procedure within 29 days of completing the radiation treatment.
Low-Activity Implants
Preoperative imaging is used to determine the volume of the resection cavity that will need to be implanted after surgery. After the surgeon has communicated to the radiation oncologist the anticipated extent of resection, the radiation oncologist estimates the number of seeds and total activity required to deliver the desired dose over the lifetime of the permanent sources, assuming 1-cm2 spacing on the walls of the resection cavity. An image-guided craniotomy for resection is then performed, after which the resection wall must exhibit no signs of bleeding without any covering of hemostatic agents such as Surgicel. The surgeon and radiation oncologist then don radioprotective garments, and the surgeon places individual sterile 125I seeds on or in the walls of the resection cavity at 1-cm2 spacing. The radiation oncologist and surgeon count the number of sources. The surgeon can use fibrin glue to secure the seeds in position. Once the patient’s skin is closed, metered readings of radiation output are taken 1 m from each side of the patient’s head. Computed tomography (CT) can be performed postoperatively to visualize the seeds in the resection cavity (Fig. 253-3), and the radiation oncologist can generate a diagram of dose/isodose levels overlaid on the postoperative CT scan (Fig. 253-4).
Intracavitary Brachytherapy
Either the patient is placed in a stereotactic frame and cranial imaging is performed, or for a frameless approach, Mayfield pins are used and preoperative MRI is registered with an image guidance system. Cyst volume is calculated from the preoperative images. The amount of 32P, the only isotope approved for intracavitary brachytherapy in the United States, needed to deliver 200 Gy to the cyst wall can then be calculated with a method described elsewhere.21 A bur hole is placed over a safe entry point identified by the surgeon, and the cyst is then stereotactically punctured with a needle guided to a target within the cyst by standard frame-based or frameless methods. The central stylet is removed, and a volume of cyst fluid is removed and measured. The 32P is injected, and any remaining isotope is then flushed out of the needle with a volume of sterile saline equal to the volume of cyst fluid removed minus the volume of 32P already injected. The needle and syringes used are collected by the radiation technologist, and the wound is closed in the usual manner.
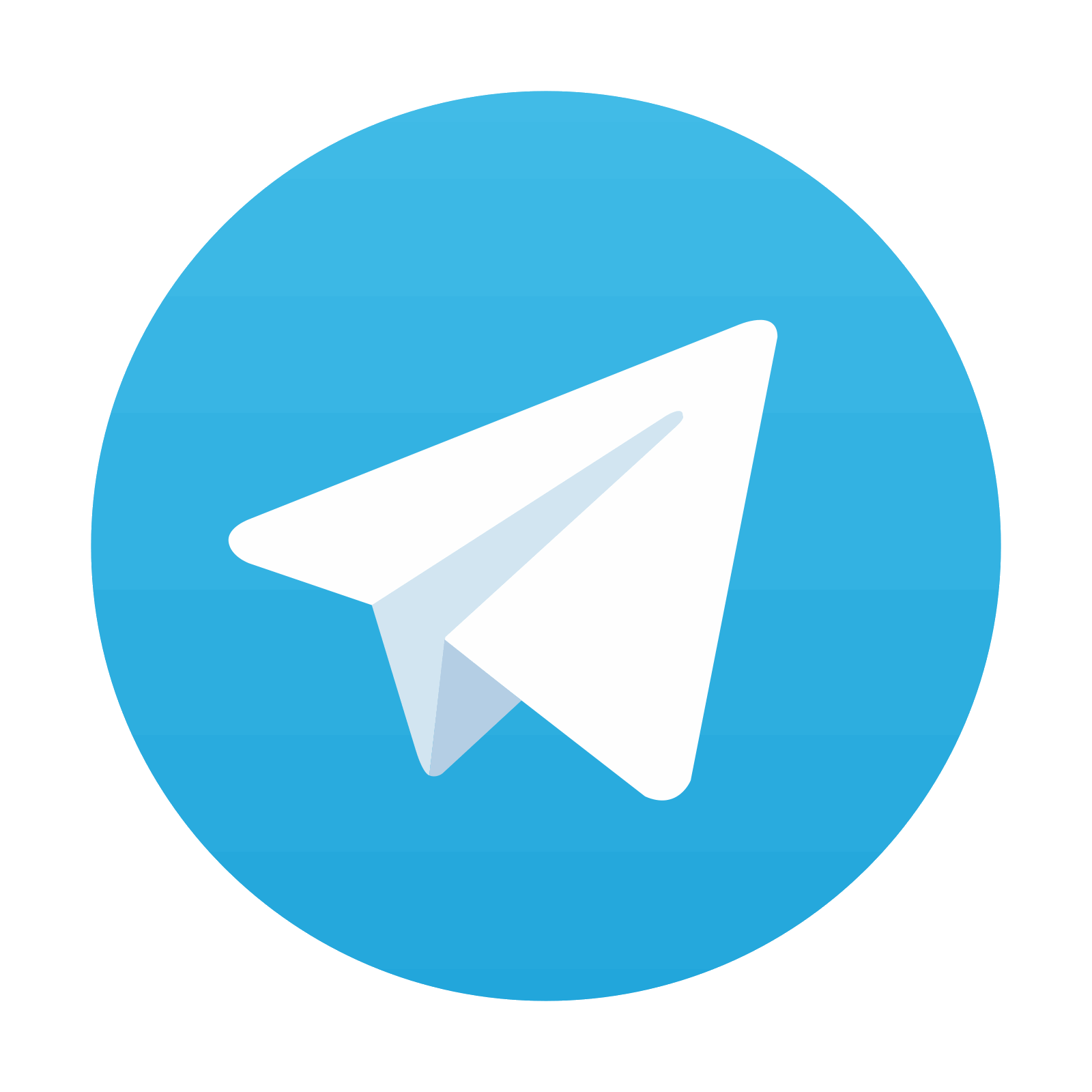
Stay updated, free articles. Join our Telegram channel
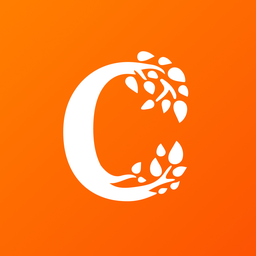
Full access? Get Clinical Tree
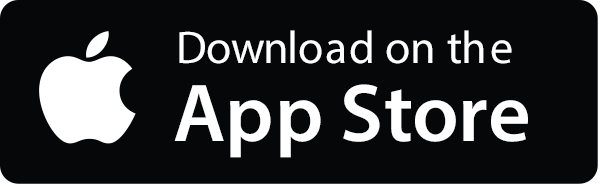
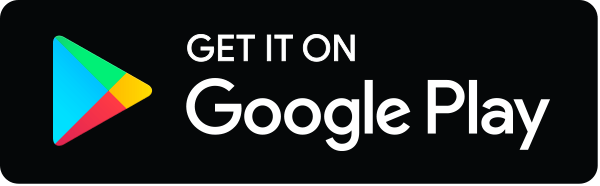