Intraoperative Neurophysiological Monitoring
The intraoperative identification of neural structures by electrophysiologic means (i.e., “mapping”) has been used for many years in a provocative manner to guide the surgical procedure. Much of our understanding of the functional role played by specific regions of the cerebral cortex in humans came from examining the effects of electrical stimulation applied to the exposed cerebral cortex in awake humans.1 However, intraoperative monitoring is also developing an important niche in neuroendovascular procedures, particularly in patients requiring general anesthesia during provocative testing for arteriovenous malformation (AVM) embolization and occlusive procedures such as balloon angioplasty or balloon-assisted aneurysm coiling. Intraoperative monitoring allows the proceduralist to detect changes in neurological function when an exam cannot be obtained, and it can also be used to prevent permanent neurological injury in some situations.
In the broadest sense, intraoperative neurophysiological monitoring (IOM) has two main applications: (1) mapping, both to identify structures and to evaluate the proximity of certain implants to nearby neural structures and (2) neuroprotection, to guard against inadvertent (i.e., iatrogenic) injury to neural structures as a direct consequence of surgical or interventional activities.
In its arguably more widely practiced role, IOM is also used for neuroprotection, to provide information about the functional status of central nervous system (CNS) structures during procedures that place these structures at risk. Should a surgical or endovascular action, such as provocative Brevital testing prior to AVM embolization, lead to a change in IOM-specific waveforms, the surgical team is alerted to this change, giving them the opportunity to undo or avoid that action. This second role of IOM—to provide immediate feedback about CNS functional integrity in the anesthetized (or comatose) patient—will serve as the focus of the remainder of this chapter.
It is important at the earliest stage of this discussion to point out that an assumption at the heart of IOM for neuroprotection is that a changed waveform reflects functional deterioration within the neurologic pathway mediating that waveform. Thus, if the IOM test being used does not utilize that portion of the CNS at risk from the procedure, then the test will not be useful in predicting injury. In the following sections, we will provide an overview of the types of IOM tests that are now considered standard in the field, and the pathways and relevant vascular supplies that are monitored by these tests.
Electroencephalogram (EEG)
EEG—Signal Generators and Pathways
The electroencephalogram (EEG) reflects the spontaneous and time-varying post-synaptic activity of large populations of neurons within the cerebral cortex. In the healthy individual, there is an inverse relationship between the frequency of EEG activity and its amplitude, as summarized in Table 11.1. A reduction in mean EEG can be perfectly normal (e.g., in a person shifting from waking toward sleep), or it may reflect a more sinister cause, such as inadequate cortical oxygenation due to hypoperfusion or diminished oxyhemoglobin.
For IOM, either raw or processed (fast-Fourier transformed) EEG is continually sampled from multiple recording sites on the scalp. Sudden changes in this spontaneous activity may reflect the consequences (usually vascular in nature) of some surgical action and serve as one of the primary outcome measures for this type of monitoring.
The EEG originates from populations of neurons in cortical and subcortical regions, hence this approach examines functional activity within gray matter rather than projection tracts. For extracranial electrodes (that is, those on or just under the scalp), the most superficial 3–5 mm of cortex contributes most of the signal power. For IOM applications, a minimal series of electrodes would include sites over the frontal, temporal, and parietal lobes bilaterally.
EEG—Vascular Considerations
The distinctions between regional arterial blood supply are highly relevant to this review, as it means that regions giving rise to upper-limb versus lower-limb motor and sensory cortices have dissimilar blood supplies. These vascular distributions are reviewed in Chapter 1 and summarized in Table 11.2.
EEG—Methods
EEG is used during IOM in one of two ways. One can look for changes in stable levels of EEG activity as an indicator of potential insult to the cerebral cortex. Alternatively, one can deliberately depress EEG activity pharmacologically, thereby lowering cortical metabolic demand in anticipation of, or in response to, an interruption of cortical blood flow.
During induction of anesthesia, the EEG will demonstrate a characteristic pattern of progressively lower mean frequency content, with superimposition of brief, larger-amplitude spikes, bearing some resemblance to certain stages of sleep. At even deeper levels of anesthesia, the EEG will begin to oscillate between prolonged periods of very limited activity (isoelectric) and shorter periods (or “bursts”) of activity. This stage is known as burst-suppression.
Virtually all general anesthetics will reduce EEG frequency and its total amount (that is, its power) once a steady-state has been achieved; at higher concentrations, some can even cause an isoelectric state. Given this, it is important that in the event of a significant EEG change, anesthetic considerations are ruled out before the endovascular team is alerted to this change. Note that some anesthetic agents, including etomidate and propofol, may result in a brief stage of increased cortical spiking activity during induction, which may be accompanied by myoclonic-like jerks or tremors in the extremities. Such activity can be confused with a seizure, but it is thought to reflect a transient imbalance between the anesthetic depth of cortical versus subcortical motor areas.2
EEG—Indications
The establishment of EEG burst-suppression via thiopental renders cortical neurons less susceptible to ischemic injury.2 Not all hypnotic agents are equal in this neuroprotective role.2 Burst-suppression is routinely called for by many physicians as a prophylaxis during procedures in which the patient’s cerebral oxygenation might be compromised, such as when the common carotid artery is cross-clamped during endarterectomy (Fig. 11.1).3
EEG changes associated with cortical ischemia during neurovascular procedures are often unilateral. In contrast, a major change in systemic physiology, like the delivery of a bolus of anesthetic agent or profound hypothermia, will affect both cortical hemispheres in parallel. A unilateral change in EEG normally serves as a reliable signal that neuronal injury might be underway, necessitating a warning to the surgical team (Table 11.2).
Prophylactic use of burst-suppression prior to initiation of a high-risk procedure may mask important changes in the EEG that reflect compromised cerebral perfusion. We recommend against the use of burst-suppression unless there is evidence of an acute change in the EEG that is suggestive of altered (that is, diminished) oxygenation of the cortical tissue giving rise to that EEG signal. This way, the sensitivity of the EEG to change would be maintained at its highest level, yet the neuroprotective effects of burst-suppression could be implemented shortly after a detection of a change in EEG characteristics.
A different circumstance in which one looks for a brief and total abolishment of EEG activity is encountered during procedures to treat vascular malformations (pial arteriovenous malformation or dural arteriovenous fistula) of the brain. In this case, cortical tissue and the malformation may share a common arterial supply, so that one would not normally choose to embolize the malformation because of a concern for a worsening of neurologic function. To establish the functional link—if any—between the vascular malformation and cortical tissue, an endovascular catheter can be placed with its tip at the malformation’s nidus. A bolus of relatively short-acting barbituate (typically sodium amobarbital or methohexital) is injected into the arterial feeding pedicle of the malformation. If the level of EEG power from nearby electrodes shows a marked decline, this indicates that the malformation includes a branch to the cerebral cortex, and it should therefore be left intact to prevent an ischemic complication. This approach takes its origins from the Wada test, which is used to localize eloquent cortex for speech and memory during craniotomy procedures when the patient is awake.3
EEG—Strengths and Weaknesses
One great strength of EEG as a monitored outcome measure for IOM techniques—its simplicity—also serves as a weakness, in that the EEG provides a sense of the neurologic status of just the cerebral cortical layers of the CNS, primarily from those gyri that are adjacent to the skull (Table 11.2).
EEG—Risks
The risks directly associated with EEG monitoring are relatively low (Table 11.2).
EEG—Anesthesia
Several reviews cover many aspects of neuroanesthesia, including intraoperative monitoring.4,5 We will simply remind the reader that virtually all agents used to sedate or induce general anesthesia in patients will cause a reduction in EEG power once a steady state has been established. Several commercially available devices (such as the BIS monitor) have been developed to quantify EEG to predict various stages (or “depths”) of sedation. More in-depth examinations of these technologies can be found elsewhere.6
Evoked Potentials
General Comments
A variety of different evoked potentials are available to the monitoring specialist (see Table 11.2). In general the idea is to activate nerve cells on one side of the surgery (“upstream”), causing electrical signals within these nerve cells to conduct through and beyond the region at risk from the surgery, and then to record the response to this activation from the same (rarely) or a different (usually) population of nerve (or muscle) fibers that are “downstream” from the surgery site. If the signals downstream from where surgery is taking place are unchanged, this indicates that the nerves conducting that signal have not been affected by the surgery. Provided certain confounding factors have been ruled out (examples to follow), the team is immediately alerted and will attempt to undo surgically whatever led to that signal change, to reduce the risk of permanent neural injury.
Somatosensory Evoked Potential (SEP)
SEP—Signal Generators and Pathways
Somatosensory evoked potentials typically use electrical stimulation of the median or ulnar nerves at the wrist or of the posterior tibial nerve at the ankle. A single stimulus activates large sensory fibers, whose action potentials are conducted proximally to the spinal cord and up the dorsal columns to the brainstem nuclei (gracile or cuneate). After synapsing, second-order fibers decussate at the level of the medulla as internal arcuate fibers and travel via the medial lemniscus to the ventroposterolateral thalamus, the site of a second synaptic relay. Finally, a third group of fibers from the thalamus transmits this nerve volley via the internal capsule to the postcentral gyrus of the parietal lobe’s primary somatosensory cortex, which forms the final destination of these nerve signals.
SEP—Vascular Considerations
In the spinal cord, the dorsal columns and related neuronal structures are supplied by the posterior spinal arteries (PSA) arising from the vertebral arteries (VA) and are reviewed in Chapter 2 and Table 11.2.
SEP—Methods
With appropriately placed electrodes, one can follow the SEP signal from its origin (the wrist or ankle) all the way to the contralateral cerebral cortex. While surface EEG cup-type electrodes can be used for recording, it is far more common in the operating room setting to use needle electrodes for EEG and evoked potential applications (usually corkscrew electrodes, because they are virtually impossible to dislodge accidentally).
Stimulation to the nerve must be of sufficient intensity to cause action potentials in the large myelinated fibers within that mixed nerve. Adequate stimulation can be confirmed by brisk contractions of intrinsic muscles in the hand and foot, provided that neuromuscular blockade is not in place. Despite the fact that the stimulus intensities suggested above typically exceed the maximum needed to activate all large myelinated fibers in that nerve (that is, it is supramaximal), the resultant SEP is too small to see, so that signal averaging is necessary to generate a reliable SEP reading. In practice, one typically averages 200 to 500 individual trials (the actual number depends on the amount of background noise). This means that the results of an SEP test will be delayed one to three minutes. We will warn the procedural team if the major component of the waveform amplitude declines by more than 50% (peak-to-peak) of the baseline or if the latency to the initial peak/trough in that waveform increases by more than 10%.
SEP—Indications
Any procedure (surgical or endovascular) that places the pathways outlined earlier at risk is a candidate for SEP monitoring (see Table 11.2). For vascular cases, SEPs are useful for establishing the functional consequences of vascular perturbations. Often this testing can be done in a provocative manner, whereby application of a temporary clip or provocative intra-arterial testing can be used and the SEPs evaluated for change. If no change in waveforms develops over the next four to eight minutes, this indicates that the neural pathways mediating that SEP either are not perfused by the blood vessel in question or are receiving adequate flow from collateral sources.7
SEPs can be used to confirm that the somatosensory pathway to the cerebral cortex is still functional, even during periods of deep anesthesia, as demonstrated by a burst-suppression pattern (as illustrated in Fig. 11.3B). However, further depression of cortical activity, either through still-deeper anesthesia or because of ischemia, will soon lead to the loss of even the SEP.
SEP—Strengths and Weaknesses
The SEP test procedure is relatively quick and simple to administer, and is well tolerated by most awake subjects (Table 11.2
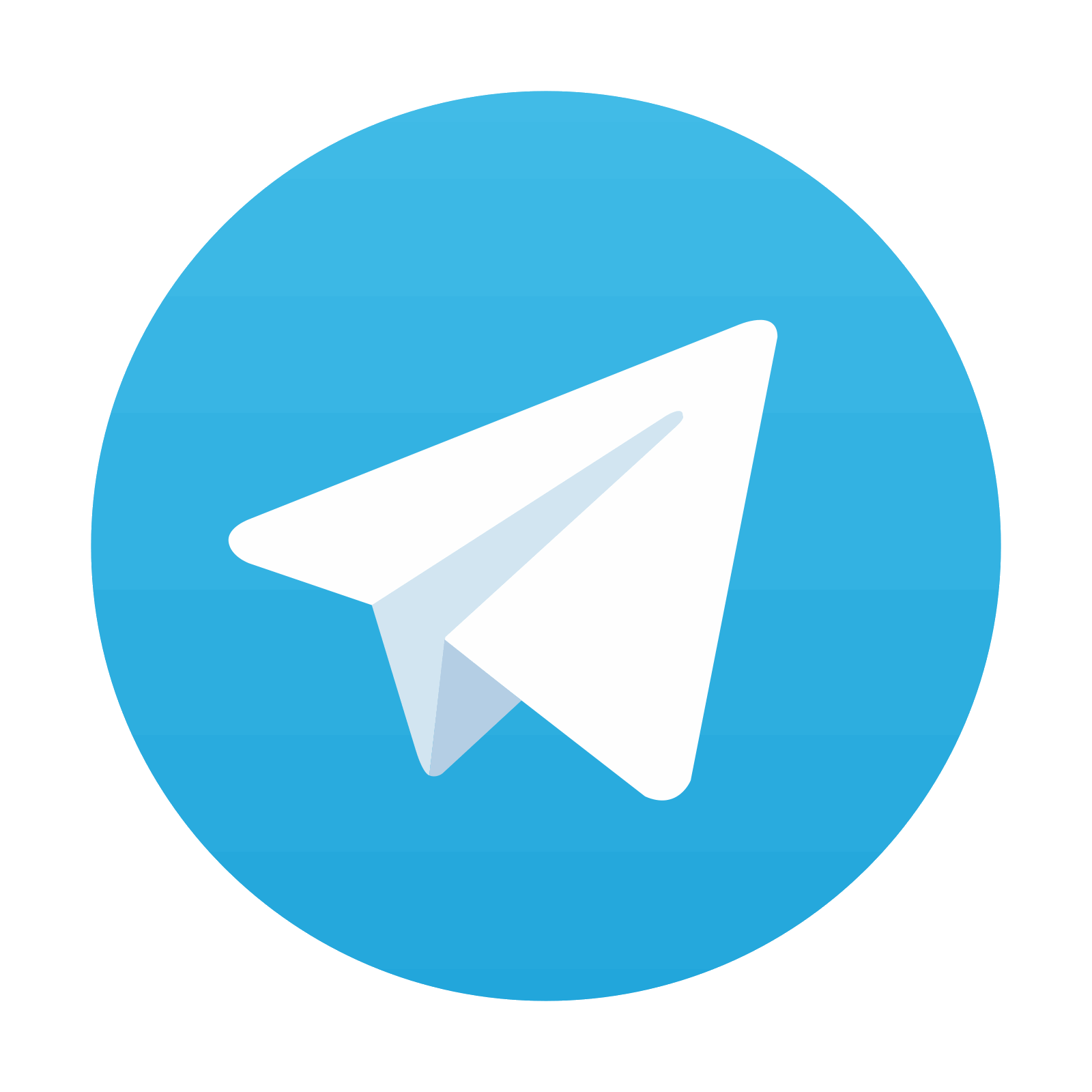
Stay updated, free articles. Join our Telegram channel
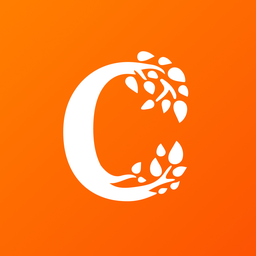
Full access? Get Clinical Tree
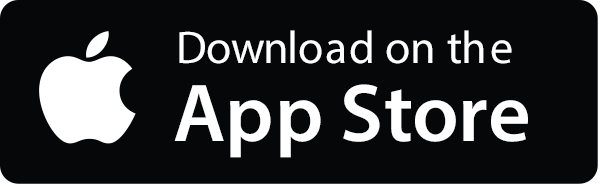
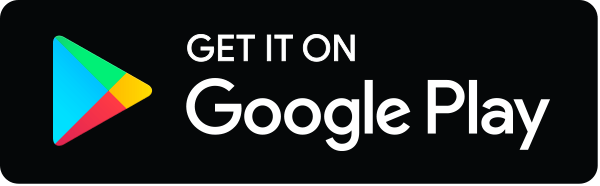