Keywords
Fatty acid oxidation, lipid storage myopathies, recurrent myoglobinuria
Historical Background
Defects in fatty acid oxidation (FAO) are an important group of disorders because they are potentially rapidly fatal and a source of major morbidity. They encompass a spectrum of clinical disorders including progressive lipid storage myopathy, recurrent myoglobinuria, neuropathy, progressive cardiomyopathy, recurrent hypoglycemic hypoketotic encephalopathy or Reye-like syndrome, seizures, and mental retardation. All of the known conditions are inherited as autosomal recessive diseases, and there often is a family history of sudden unexpected death or SIDS (sudden infant death syndrome) in siblings. Early recognition and prompt institution of therapy and preventative measures, and in certain cases specific therapy, may be life-saving, significantly decreasing long-term morbidity, particularly with respect to central nervous system sequelae.
Engel identified the first genetic defect in FAO in 1970 when he described a skeletal muscle disorder associated with intermittent symptoms and a possible defect in lipid metabolism. DiMauro and DiMauro reported the first enzyme defect in 1973; namely, carnitine palmitoyltransferase (CPT) deficiency, which presented with recurrent myoglobinuria. There are now at least 16 recognized enzyme or transporter defects in FAO, most of which have been diagnosed in the last 25 years. With the significant advances in biomedical technology, there has been a rapid increase in the number of subsequently diagnosed cases, for example of MCAD deficiency. The incidence for MCAD deficiency was found to be 1 in 8930 live births in a survey from the Pennsylvania newborn screening program using tandem mass spectrometry, followed by confirmation through molecular analysis for several common mutations. Similarly the incidence of MCADD detected by recent newborn screening using MS/MS and confirmed by mutation analysis was 1/8954 in Denmark. The spectrum of FAOD differs widely among ethnic groups. Incidence calculations from a total of 5,256,999 screened newborns from the USA, Australia, and Germany give a combined incidence of all FAOD of approximately 1:9300.
The delay in recognition of these disorders can be attributed to three major factors. First, FAO does not play a major role in energy production until relatively late in fasting. Thus, affected individuals may remain clinically silent until they are exposed to periods of fasting beyond 12 hours or to prolonged exercise. Acute decompensations may be precipitated by an intercurrent infection with fasting, vomiting, and shivering thermogenesis. Secondly, routine laboratory tests, such as urinary ketones, may not demonstrate the defect in FAO, unless the blood and urine samples are obtained at the time of the acute episode. If they are obtained after the child has received intravenous glucose therapy or has recovered from the acute illness, the defect may be missed. Thirdly, there has been a rapid expansion in the methodologies to identify defects in FAO such as the introduction of acylcarnitine analysis by tandem mass spectrometry in newborn screening; nevertheless there remains a need to unify confirmatory diagnostic procedures such as specific enzyme assays. Mutation analysis may confirm diagnosis and may also help to explain differences in phenotypic expression in patients with the same enzyme defect. Different mutations may have different effects on protein function, as certain missense mutations may result in misfolded but stable proteins that may affect the integrity and function of the FAO enzyme versus pure null mutations that lead to absence of the protein. Gene-gene and environmental factors may also influence phenotypic expression of a given genotype.
An overview will be given of the FAO pathway, its enzymes, and the role of carnitine, as well as a discussion of normal fasting adaptation in human physiology to lay the groundwork for understanding the pathophysiology of these diseases. This will be followed by the clinical and laboratory features of specific FAO defects, an approach to diagnosis, and current treatments.
Pathophysiology
Normal Pathway of Fatty Acid Oxidation
The pathway of mitochondrial FAO is outlined in Figure 40.1 . During fasting, free fatty acids are liberated from adipocytes and are transported to other tissues either as triglyceride-rich lipoproteins or bound to serum albumin. Triacylglycerols are hydrolyzed outside the cells by lipoprotein lipase to yield free fatty acids (FFAs). The mechanism of FFA entry into cells is not well understood; however, there is kinetic evidence to suggest both a saturable and nonsaturable uptake of FFAs. Once across the plasma membrane, short- (e.g. 4-carbon) and medium-chain (e.g. 8-carbon) fatty acids (less than 10 carbon atoms) are able to cross the outer and inner mitochondrial membranes as free acids to enter the mitochondrial matrix. Here they are then activated by their respective short- and medium-chain acyl-CoA synthetases to their CoA thioesters for ensuant intramitochondrial β-oxidation.

The mitochondrial membrane is impermeable to long-chain fatty acids (e.g. 16-carbon). Therefore, long-chain fatty acids diffuse or are transported to the outer mitochondrial membrane and to the endoplasmic reticulum. At both locations they are activated by conversion to their CoA thioesters. Long-chain acyl-CoA synthetase is a membrane-bound enzyme located in the endoplasmic reticulum and in the outer mitochondrial membrane. This enzyme acts on saturated fatty acids containing 10 to 18 carbon atoms and on unsaturated fatty acids containing 16 to 20 carbon atoms. Most of the activated fatty acids are directed toward mitochondrial β-oxidation. The inner mitochondrial membrane is impermeable to CoA and its derivatives, namely fatty acyl-CoA thioesters, which have been formed at the outer mitochondrial membrane. Thus these thioesters cannot directly enter the mitochondrial matrix. Therefore, the long-chain acyl-CoA must first be converted into its acylcarnitine form, e.g. palmitoylcarnitine, with release of free CoA. This is accomplished by the reversible enzyme carnitine palmitoyltransferase I (CPT I), which uses carnitine as a cofactor and is located on the inner side of the outer mitochondrial membrane. The palmitoylcarnitine is then translocated across the inner mitochondrial membrane by carnitine:acylcarnitine translocase which catalyzes a slow unidirectional diffusion of carnitine both in and out of the mitochondrial matrix in addition to a much faster mole-to-mole exchange of acylcarnitine for carnitine, carnitine for carnitine, and acylcarnitine for acylcarnitine. In the mitochondrial matrix, carnitine palmitoyltransferase II (CPT II), which is situated on the inner side of the inner mitochondrial membrane, converts palmitoylcarnitine, in the presence of free CoA, back to palmitoyl-CoA and carnitine.
There are four sequential steps in mitochondrial β-oxidation, which are composed of chain-length specific enzymes ( Figure 40.2 ). It was originally believed that the complete intramitochondrial oxidation of long-chain fatty acids required a minimum of nine enzymes including the three genetically distinct acyl-CoA dehyrogenases (short-, medium-, long-chain) and at least two enoyl-CoA hydratases (crotonase and long-chain enoyl-CoA hydratase), two L-3-hydroxyacyl-CoA dehydrogenases (short- and long-chain) and two 3-ketoacyl-CoA thiolases (acetoacetyl-CoA thiolase and generalized 3-ketoacyl-CoA thiolase). Further enzymatic and protein characterization has revealed the presence of an additional very-long-chain acyl-CoA dehydrogenase enzyme, of an acyl-CoA dehydrogenase 9 (ACAD9) that demonstrates maximal activity with unsaturated long-chain acyl-CoAs, and of a trifunctional protein which combines the activities of the long-chain enoyl-CoA hydratase, long-chain-L-3-hydroxyacyl-CoA dehydrogenase, and the long-chain thiolase enzymes. These enzymes are chain-length specific. For example, the long-chain acyl-CoA dehydrogenase has specificity for 12–18 carbon fatty acids and the medium-chain acyl-CoA dehydrogenase has specificity for 4–12 carbon fatty acids. Despite a significant overlap of substrate specificity, it appears that ACAD9 and VLCAD are unable to compensate for each other in patients with either deficiency. Studies of the tissue distribution and gene regulation of ACAD9 and VLCAD identify the presence of two independently regulated functional pathways for long-chain fat metabolism, indicating that these two enzymes are likely to be involved in different physiological functions. ACAD9 is required for the assembly of mitochondrial complex I. ACAD9 mutations result in complex I deficiency without disturbing long-chain fatty acid oxidation. This strongly contrasts with its evolutionary ancestor VLCAD, which is not required for complex I assembly and clearly plays a role in fatty acid oxidation. The trifunctional protein (TFP) is a heterocomplex of four alpha and four beta subunits, which are encoded by two nuclear genes. The alpha subunit contains the long-chain enoyl-CoA hydratase and LCHAD activities, and the beta subunit contains the long-chain 3-ketoacyl-CoA thiolase activity. There are two different biochemical phenotypes of TFP deficiency. The first includes deficiency of all three enzyme activities of the TFP, with loss of both the alpha and beta subunits by immunoblotting studies. The second has isolated LCHAD deficiency with preservation of the long-chain enoyl-CoA hydratase and 3-ketoacyl-CoA thiolase activities and normal amounts of the alpha and beta subunits.

With each complete cycle, a 2-carbon fragment is cleaved and an acetyl-CoA moiety is released. In most tissues, such as muscle and heart, the acetyl-CoA is oxidized for energy production via the tricarboxylic acid cycle. In liver, about 90% of the hepatic acetyl-CoA is converted into ketones via the coordinated action of acetyl-CoA acetyltransferase, β-hydroxy β-methylglutaryl-CoA synthase, and β-hydroxy β-methylglutaryl-CoA lyase. These ketones are then exported for final oxidation by other tissues, such as brain. Ketolysis in extrahepatic mitochondria is mediated by two reversible reactions catalyzed by succinyl-CoA:3-ketoacid-CoA transferase (SCOT) and mitochondrial acetoacetyl-CoA thiolase.
The Regulation of Fatty Acid Oxidation
There are several levels of fatty acid oxidation regulation. The rate of FAO is determined by the availability of fatty acids and by the rate of utilization of β-oxidation products. The concentration of nonesterified fatty acids in plasma is regulated by the hormones glucagon, which stimulates, and insulin, which inhibits the breakdown of triacylglycerols in adipose tissue. Glucagon activates adenylate cyclase leading to an increase in the concentration of cellular cyclic AMP, which in turn activates protein kinase. One of the substrates of protein kinase in adipose tissue is the hormone-sensitive lipase. This lipase is activated by phosphorylation and inactivated by dephosphorylation. Therefore, when the concentration of glucose is low, as in fasting, there is a high glucagon-to-insulin ratio, which results in an increase in plasma nonesterified FFA. These fatty acids will enter cells, where they can be either degraded to acetyl-CoA or incorporated into other lipids. The utilization of fatty acids for either oxidation or lipid synthesis depends both on the nutritional state and on the availability of carbohydrates.
Because of its role in ketogenesis, the regulation of FAO in the liver differs and is more complex than the regulation in heart and skeletal muscle. In the fed state, the liver breaks down carbohydrates to synthesize fatty acids. In contrast, in the fasted animal, FAO, ketogenesis, and gluconeogenesis are more active. McGarry and Foster suggested that the concentration of malonyl-CoA, which is the specific reversible inhibitor of CPT I, determines the rate of FAO. In the fed state, where glucose is converted to fatty acids, the concentration of malonyl-CoA is elevated, thereby leading to an inhibition of CPT I activity. This inhibits the transfer of long-chain fatty acyl residues from CoA to carnitine so that long-chain acylcarnitines cannot be translocated into the mitochondria. Consequently, β-oxidation is depressed. When there is a change from the fed to the fasted state, hepatic metabolism shifts from glucose breakdown to gluconeogenesis, leading to a decrease in fatty acid synthesis. The concentration of malonyl-CoA decreases and the inhibition of CPT I is relieved whereby acylcarnitines are then formed and translocated into mitochondria for β-oxidation and ketogenesis. The cellular concentration of malonyl-CoA is directly related to the activity of acetyl-CoA carboxylase, which is hormonally regulated. In fasting, there is an increase in the glucagon:insulin ratio which causes an increase in cellular cAMP which, in turn, is responsible for the phosphorylation and inactivation of acetyl-CoA carboxylase. As a consequence, the concentration of malonyl-CoA and the rate of fatty acid synthesis decrease, while the rate of β-oxidation increases. A decrease in the glucagon:insulin ratio reverses these effects. Both fatty acid synthesis and FAO are regulated by the glucagon:insulin ratio. A third area that may play a key role in regulating FAO occurs in the mitochondria, where end-product inhibition of proximal β-oxidation by more distal acyl-CoA intermediates prevents excessive accumulation of acyl-CoAs even under conditions of very rapid FAO.
Alternative Pathways for Fatty Acid Metabolism
Under normal circumstances, mitochondrial β-oxidation accounts for the majority of fatty acid metabolism; however, there are other available mechanisms for the oxidation or disposal of fatty acid intermediates. Peroxisomes contain an oxidation pathway which is composed of enzymes that are genetically distinct from the mitochondrial enzymes. The peroxisome may contribute up to 20% of total cellular FAO under conditions of prolonged fasting. When there is an excessive accumulation of acyl-CoAs, they are shunted to microsomes where they undergo omega oxidation. This places a carboxyl group on the methyl-terminal end of the fatty acid and results in the formation of a dicarboxylic acid. Dicarboxylic acids (DCAs) are found in most conditions where the capacity for β-oxidation is exceeded. These include normal fasting, diabetic ketoacidosis, feeding with medium-chain triglycerides, and genetic defects in intramitochondrial β-oxidation. The specific pattern of DCAs found in serum or urine may be diagnostically useful in the identification of specific inborn errors of FAO.
Three additional mechanisms may be important when there is an impairment in mitochondrial β-oxidation. These include the conjugation of acyl groups to glycine and to carnitine and the deacylation of CoA by thioesterases. These glycine and carnitine conjugates are able to cross cellular membranes more readily than the respective CoA ester. This prevents excessive accumulation of intracellular acyl-CoAs and is important because acyl-CoA esters inhibit specific enzymes and transporters. Removal of intracellular acyl groups also preserves free CoA for other enzymes which require CoA as a cofactor. Measurement of acylcarnitines and acylglycines is diagnostically useful in the identification of genetic defects in FAO.
Central Role of Carnitine Metabolism
Carnitine (beta-hydroxy-gamma-trimethylaminobutyric acid), a water-soluble quartenary amine, has several important intracellular functions : (1) it modulates the intramitochondrial acyl-CoA/CoA sulfhydryl ratio in mammalian cells; (2) it serves as an essential cofactor for mitochondrial FAO by transferring long-chain fatty acids as acylcarnitine esters across the inner mitochondrial membrane; (3) it facilitates branched-chain alpha-keto acid oxidation; (4) it shuttles acyl moieties that have been chain-shortened by beta-oxidation out of peroxisomes in the liver; (5) it traps potentially toxic acyl-CoA metabolites that may increase in excess during acute metabolic crises through esterification to carnitine. These metabolites may secondarily impair the citric acid cycle, gluconeogenesis, the urea cycle, and fatty acid oxidation.
In omnivores, approximately 75% of carnitine sources comes from the diet with 25% from endogenous biosynthesis. The principal dietary sources include meat, poultry, fish and dairy products. Approximately 70–80% of dietary carnitine is absorbed in omnivores, whereas in strict vegetarians, endogenous carnitine synthesis provides more than 90% of total available carnitine. There are adequate carnitine concentrations in human milk and most supplemented milk-based formulas to sustain early growth and development. The plasma carnitine concentrations were found to be markedly reduced in term infants fed unsupplemented soy protein-based formulas compared to those in supplemented infants. Skeletal muscle is the major tissue reservoir of carnitine, containing over 90% of total body carnitine stores. Under normal conditions, the carnitine concentration in tissues, other than brain, is 20- to 50-fold higher than in plasma and parallels the capacity of the tissue to metabolize fatty acids; human tissue concentrations (nmol/g) are heart (3500–6000)>muscle (2000–4600)>liver (1000–1900)>brain (200–500). Thus, plasmalemmal carnitine uptake occurs across a large concentration gradient which is maintained by a transport system that is generally held to be sodium-gradient and energy-dependent. The normal serum carnitine concentration is tightly maintained by the renal threshold, which is 40 μmol/L. The kidney is capable of adjusting to wide variations in dietary carnitine as carnitine is not significantly degraded in the body. More than 90% of filtered carnitine is reabsorbed by the kidney at normal physiological plasma carnitine concentrations. Human skeletal muscle, heart, liver, kidney and brain are capable of the biosynthesis of carnitine from methionine and lysine to its immediate precursor gamma-butyrobetaine. However, the final conversion of gamma-butyrobetaine to L-carnitine by gamma-butyrobetaine hydroxylase can only be done in liver, kidney, and brain. Gamma-butyrobetaine is thus exported to these tissues for final conversion to L-carnitine. Hepatic gamma-butyrobetaine hydroxylase is developmentally regulated, being approximately 25% of adult activity at birth.
Since 1973, many patients with carnitine deficiency have been described. Originally they were divided into two groups: those with a systemic form characterized by recurrent coma with low carnitine concentrations in serum, liver and muscle versus a muscular form characterized by a progressive lipid storage myopathy in which the serum concentrations were normal and the carnitine deficiency was confined to skeletal muscle. However, with recent advances in biomedical technology, many of these cases were found to be due to intramitochondrial β-oxidation defects with secondary carnitine deficiency . For example, many cases of the systemic form were found to be due to medium-chain acyl-CoA dehydrogenase (MCAD) deficiency. Similarly, certain cases of the myopathic form were attributed to short-chain acyl-CoA dehydrogenase (SCAD) deficiency. The secondary carnitine deficiency disorders can be divided into (1) genetic, (2) acquired, and (3) iatrogenic forms ( Box 40.1 ).
Genetically Determined Metabolic Errors
Increased Esterification due to acyl-CoA Accumulation
Carnitine acyl-carnitine translocase deficiency
Carnitine palmitoyltransferase II deficiency
Very long-chain acyl-CoA dehydrogenase deficiency
Long-chain acyl-CoA dehydrogenase deficiency
Long-chain L-3-hydroxyacyl-CoA dehydrogenase deficiency
Trifunctional protein deficiency
Medium-chain acyl-CoA dehydrogenase deficiency
Short-chain acyl-CoA dehydrogenase deficiency
Short-chain L-3-hydroxyacyl-CoA dehydrogenase deficiency
Multiple acyl-CoA dehydrogenase (ETF and ETF/Qo) deficiency
beta-Hydroxy-beta-methylglutaryl-CoA lyase deficiency
Isovaleric acidemia
Propionic acidemia
Methylmalonic aciduria
Glutaryl-CoA dehydrogenase deficiency (glutaric aciduria type I)
β-ketothiolase deficiency
Decreased Biosynthesis
Homocystinuria
5-methylene tetrahydrofolate reductase deficiency
Adenosine deaminase deficiency
Ornithine transcarbamylase heterozygote state
Increased Urinary Loss
Cystinosis
Cytochrome oxidase deficiency
Acquired Medical Conditions
Decreased Biosynthesis a
a often combined factors.
Cirrhosis
Chronic renal disease
Extreme prematurity
Dietary Deficiency a
Chronic TPN without carnitine supplementation
Malabsorption (cystic fibrosis, short-gut syndrome)
Unsupplemented Soybean protein-derived infant formula
Decreased Body Stores
Extreme prematurity
Intrauterine growth retardation
Infant of carnitine-deficient mother
Increased Urinary Loss
Fanconi syndrome
Renal tubular acidosis
Iatrogenic Factors: Drug Therapy
Increased Esterification and competitive inhibition of carnitine uptake by valproylcarnitine
Chronic valproic acid administration
Impaired Hepatic Biosynthesis
Chronic valproic acid administration
Increased Loss
Chronic hemodialysis
Fasting Adaptation
Fats comprise the most important and efficient fuel for oxidative metabolism. The largest reserve of fuel in the body is comprised of fatty acids stored as adipose tissue triglyceride. As liver glycogen stores are depleted within a few hours of a meal and since there are no reserve stores of protein in the body, fatty acids become the predominant substrate for oxidation quite early in fasting. In adults, approximately 80% of caloric requirements after a 24-hour period of fasting is supplied by fatty acids, which increases to 94% during more prolonged fasting. Fatty acids serve three major functions. First, the partial oxidation of fatty acids by the liver produces ketones (acetoacetate, β-hydroxybutyrate) which are an important auxiliary fuel for almost all tissues and particularly brain, as the blood-brain barrier prevents the direct use of long-chain fatty acids by the brain. This, therefore, provides an important mechanism to spare glucose oxidation and proteolysis during prolonged fasting. Second, fatty acids serve as a major fuel for cardiac and skeletal muscle. Resting muscle depends mostly on FAO. Depending upon the type, intensity, and duration of exercise, energy in working muscle is derived from either the combination of triglyceride and stored glycogen or the combination of glucose and free fatty acids. After 90 minutes, the major fuels are glucose and free fatty acids. During 1–4 hours of mild to moderate prolonged aerobic exercise, free fatty acid uptake by muscle increases by 70% and after 4 hours, free fatty acids are used twice as much as carbohydrate sources. Third, the high rates of hepatic gluconeogenesis and ureagenesis needed for maintaining fasting homeostasis are sustained by the production of ATP, reducing equivalents (nicotinamide adenine dinucleotide, reduced, +H+), and metabolic intermediates (acetyl-coenzyme A) derived from FAO.
Increased Susceptibility of the Child
Infants and young children have an increased risk of problems with fasting adaptation for several reasons. First, infants have a larger brain compared with body size which is highly dependent upon glucose and has a high rate of metabolism. Thus, infants and children show an even earlier activation of FAO with hyperketonemia within 12 to 24 hours of fasting. Second, basal energy needs in the infant are high in order to maintain body temperature, given their large ratio of surface area to mass. Their body temperature is maintained by shivering thermogenesis, which is highly dependent upon efficient FAO. Third, there is a lower activity of several key enzymes involved in energy production in the infant compared to the older child and adult, which leads to further impairment of the infant’s ability to maintain glucose homeostasis.
Clinical and Biochemical Features of Identified Defects
There are now at least 16 identified defects in fatty acid oxidation. They share a number of clinical features which suggest an underlying defect in fatty acid oxidation. These individual defects can be differentiated on the basis of distinct clinical and biochemical features. In the following sections, the common features will be presented first, followed by a discussion of the differentiating clinical and laboratory features.
Common Features of Fatty Acid Oxidation Disorders
It has been suggested that there are at least four clinical and laboratory features that should lead the clinician to suspect a genetic defect in fatty acid metabolism ( Box 40.2 ). These common features include: (1) acute metabolic decompensation in association with fasting; (2) chronic involvement of tissues highly dependent upon efficient fatty acid oxidation (e.g. muscle, heart, liver); (3) recurrent episodes of hypoketotic hypoglycemia; (4) alterations in the quantity of total carnitine or in the percentage of esterified carnitine in plasma and tissue.
- 1.
Metabolic decompensation during fasting, infection, prolonged exercise, cold exposure, and stress
- a.
Decreased oral intake, increased energy expenditure
- b.
Progressive obtundation leading to coma
- c.
Reye-like syndrome
- d.
Sudden infant death syndrome or “near-miss” SIDS
- e.
Recurrence, familial occurrence
- a.
- 2.
Involvement of fatty acid-dependent tissues
- a.
Myalgia, exercise intolerance, myoglobinuria, hypotonia, weakness
- b.
Cardiac hypertrophy/dilatation, endocardial fibroelastosis, arrhythmias
- c.
Hepatomegaly and hepatic dysfunction
- d.
Fatty infiltration of tissues on biopsy or autopsy
- a.
- 3.
Hypoketotic hypoglycemia
- a.
Elevated serum free fatty acids with serum free fatty acid to ketone body ratio >2:1
- b.
Hyperinsulinism and hypopituitarism ruled out (mandatory)
- a.
- 4.
Alterations in plasma or tissue carnitine concentration
- a.
Decreased total carnitine concentration (10–50% of normal) with increased esterified fraction in intramitochondrial fatty acid oxidation defects
- b.
Marked decrease in total carnitine concentration (<5% of normal) with normal esterified fraction in plasmalemmal carnitine transporter defect
- c.
CPT I deficiency is exception with high to normal carnitine concentration and low esterified fraction
- a.
- 5.
Clinical laboratory abnormal findings
- a.
Dicarboxylic aciduria (intramitochondrial FAO defects)
- b.
Hyperammonemia
- c.
Acidosis
- d.
During myoglobinuria, increased serum creatine kinase, hyperkalemia, hyperphosphatemia, hypocalcemia, hyperuricemia and possible increased creatinine (with renal failure)
- e.
Increased alanine and aspartate aminotransferase
- a.
Metabolic Decompensation in Association with Fasting
Episodes of metabolic decompensation occur in affected individuals during conditions that place stress on the FAO pathway for fuel generation, in the context of depleted glycogen and glucose reserves. These stressors include fasting, prolonged exercise (>1 hour of mild to moderate aerobic exercise), infection with vomiting, and cold-induced shivering thermogenesis. Shivering is an involuntary form of muscle activity which depends on long-chain fatty acid oxidation. Thus, ketogenesis is stimulated in normal individuals during cold exposure. Children are most likely to be found comatose in the early-morning hours after an overnight fast. Infections such as a viral illness are frequent precipitants due to the synergistic combination of vomiting and decreased oral intake with shivering thermogenesis. If not recognized and therefore untreated, children may progress to a Reye-like syndrome as seen in medium-chain acyl-CoA dehydrogenase (MCAD) deficiency. Infants and younger children are at greater risk during fasting than older children, because of their limited fasting adaptation capabilities. Thus, prolonged fasting for an infant of less than 1 year of age would be 6 to 8 hours, versus 12 hours for a child between 1 and 4 years of age.
Hypoketotic Hypoglycemia
The accelerated rate of glucose utilization that occurs when fatty acids cannot be used as fuels and ketone bodies are not generated to spare glucose or glycogen stores results in the pattern of hypoketotic hypoglycemia.The increase in the ratio of serum free fatty acids to ketones is another clue to a block in β-oxidation. The normal ratio should be 1:1. If this ratio exceeds 2:1, this would suggest a block in β-oxidation. Problems in fat mobilization, such as hyperinsulinism, are excluded by the increase in serum free fatty acids.
Involvement of Fatty-acid Oxidation Dependent Tissues
Tissues with high energy demands such as skeletal muscle, heart, and liver are dependent upon efficient FAO. With deficient hepatic ketogenesis, glucose becomes the only available fuel and becomes rate-limiting under conditions of FAO stress when glycogen and glucose stores have been depleted. As a result, free fatty acids, which are liberated during fasting and which cannot be metabolized due to the block, may be stored in the cytosol as triglycerides. This may lead to a progressive lipid storage myopathy with weakness as well as a hypertrophic and/or dilatative cardiomyopathy and a fatty liver, which are pathological hallmarks of genetic FAO defects. Increased short- or medium-chain fatty acids and in particular, their dicarboyxlic metabolites from compensatory omega-oxidation, may cause secondary abnormalities including an impairment of gluconeogenesis, beta-oxidation, and the citric acid cycle. This would further decrease cellular ATP production.
In addition, in the long-chain fatty acid oxidation disorders which may have recurrent episodes of acute muscle breakdown or myoglobinuria (e.g. CPT II, VLCAD, trifunctional enzyme deficiencies), and the accumulation of long-chain fatty acids (LCFAs) and long-chain acylcarnitines may have detergent-like actions on muscle membranes. Excessive amounts of palmitoyl-CoA and palmitoylcarnitine have been shown to have detergent properties on isolated canine myocytic sarcolemmal membranes and to potentiate free radical induced lipid membrane peroxidative injury in ischemia. Long-chain acylcarnitines have also been shown to activate Ca 2+ channels in cardiac and in smooth muscle myocytes and thus may potentiate the increase in cytosolic Ca 2+ associated with arrhythmogenesis as seen in ischemic myocardium.
Alterations in Plasma and Tissue Concentrations of Carnitine
The total carnitine concentration is decreased (<50 % of normal) and the acylcarnitine fraction is increased (>50% esterified; normal=10–25% in fed state and 30–50% in fasted state) in most cases of intramitochondrial β-oxidation defects. Excessive acyl-CoAs which accumulate proximal to the block, in intramitochondrial β-oxidation defects, may be converted into acylcarnitines by chain-length specific carnitine acyltransferases. When filtered through the kidneys, these acylcarnitines will compete with free carnitine at the renal tubular reabsorptive site. As demonstrated by Stanley et al. in a cultured skin fibroblast model, the longer chain-length acylcarnitines have an increasingly higher affinity for the carnitine transporter than free carnitine, and thus free carnitine will be excreted. This will with time deplete total body and muscle stores of free carnitine. In the plasmalemmal carnitine transporter defect, total carnitine is markedly reduced (e.g.<5% of normal) and the esterified fraction is normal as the transport defect in kidney leads to a decreased renal threshold for carnitine reabsorption.
Additional Laboratory Findings
A number of other biochemical abnormalities may be noted during acute catabolic crises. During the Reye-like syndrome presentation, a modest hyperammonemia (100–200 μmol/L) may be documented accompanied by 3- to 5-fold elevations of liver transaminases. With acute myoglobinuria, there are marked increases in sarcoplasmic enzymes including serum CK which may rise to >100,000 U/L (normal<250 U/L). There may also be increased serum amino acids (especially taurine), creatinine, potassium, phosphate, and urate. These changes may have deleterious effects on the kidneys and heart, increasing the damage. The hyperphosphatemia may lead to secondary hypocalcemia which may be later followed by hypercalcemia because of increased secretion of parathormone and vitamin D, with deposition of calcium in injured muscle followed by release. It is urgent to prevent renal failure. Lactic acidosis may also be noted during the acute catabolic presentations which may reflect poor perfusion or inhibition of critical enzymes such as pyruvate carboxylase by accumulated metabolites. Urine organic acid screening may demonstrate unusual compounds or excessive organic acids which may be diagnostic of a specific block in β-oxidation.
Specific Features of Individual Genetic Defects
With the exception of MCAD, the carnitine (OCTN2) transporter, classic late-onset CPT II, VLCAD, LCHAD, alpha ETF and ETF-CoQ, and HMG-CoA lyase deficiencies, there are fewer than 25 published cases for most of the FAO defects. The clinical and laboratory characteristics that are presented here are based on a summary of these cases; the phenotypes may be further broadened as additional cases are identified. Key clinical features are summarized in Table 40.1 .
Deficiency | Fasting Disorder | Tissue Involved | Hypoketotic hypoglycemia | Altered Carnitine | Dicar-boxylic acids | Reye-like syndrome | SIDS |
---|---|---|---|---|---|---|---|
LCFAUD | + | L | + | + | NR | NR | NR |
OCTN2 | + | H, M, L | + | + | NR | + | + |
CPT I Liver | + | L, K | + | + | NR a | + | NR |
TRANS | + | H, L, M, (Mg) | + | + | NR | + | + |
CPT II (mild) | ± | M, Mg, P | NR | + | NR | NR | NR |
CPT II (severe) | + | H, K, M, Mg, L,B | + | + | NR b | + | + |
VLCAD/LCAD | + | H, M, Mg, L | + | + | + | + | + |
Trifunctional/ LCHAD | + | H, M, Mg, L, N, P, R | + | + | + | + | + |
Dienoyl CoA reductase | NR | M,D,B, H | NR | + | NR | NR | NR |
MCAD | + | (Mg) | + | + | + | + | + |
SCAD | + | M, B, D, H | ± | + | + | NR | + |
SCHAD | + | H, M, Mg, L | + | + | + | NR | + |
ETF and ETF/Qo | + | M, H, K, B, D | + | + | + | NR | + |
HMG-CoA lyase | + | B, P | + | + | + | + | + ? |
a Reported in one case of infantile CPT 1 deficiency during Reye-like syndrome.
b Reported in one case of severe infantile CPT II deficiency.
There are several differentiating features. In the defects involving the transport of fatty acids into the mitochondria (plasmalemmal long-chain fatty acid uptake defect, plasmalemmal carnitine transporter defect OCTN2, CPT I, carnitine-acylcarnitine translocase, CPT II), there is generally not an associated abnormal dicarboxylicaciduria. However, this has been reported in one case of severe infantile CPT II deficiency and on one occasion during an acute metabolic decompensation in a case of infantile CPT I deficiency presenting with recurrent Reye-like syndrome. The carnitine transporter defect, carnitine acylcarnitine translocase deficiency and severe CPT II deficiency present in infancy or early childhood and involve both cardiac and skeletal muscle and recurrent episodes of hypoketotic hypoglycemic encephalopathy. The carnitine transporter OCTN2 defect is characterized by a progressive hypertrophic and/or dilatative cardiomyopathy which is pathologically similar to endocardial fibroelastosis, lipid storage limb girdle myopathy, and recurrent hypoketotic hypoglycemic encephalopathy which is exquisitely responsive to high dose oral L-carnitine supplementation, which reverses the cardiomyopathy and myopathy. Renal tubular acidosis has been documented in one case of CPT I deficiency. At this time, the only human defect that has been identified for CPT I deficiency is the hepatic and renal CPT 1A which presents with fasting-induced hepatic failure (Reye-like syndrome) with hypoketotic hypoglycemia. Notably, the P479L variant which was initially described in an Inuit individual has been identified in a large number of Alaskan Inuit and Canadian First Nations individuals. These groups have higher than normal rates of infant deaths and a significant number of newborns identified in the Alaskan newborn screening program have this DNA variant. It is uncertain whether it is a pathological mutation or an adaptive polymorphism, as the amino acid substitution removes any inhibition of CPT 1A by malonyl-CoA theoretically allowing an individual to slowly and continuously generate energy via ketone body production from fatty acids even when not fasted, which may be an adaptive advantage in the Inuit during long winter months when food is scarce and provided there are ample fat stores generated during the summer season. Fasting in the absence of adequate fat stores as in the infant may lead to the high infant mortality rate. One individual with atypical symptoms and homozygosity for P479L had ~20% normal L-CPT1 activity and presented with adult onset recurrent muscle cramps and vomiting with elevated serum CK (2733 U/L). Classic adult CPT II deficiency presents with adolescent onset recurrent episodes of acute myoglobinuria precipitated by prolonged exercise or fasting, in which power between episodes is normal and in which lipid accumulation in muscle is noted only under conditions of fasting and prolonged exercise. Fasting ketogenesis is generally normal in this condition, though it may be delayed. There is no fixed cardiomyopathy, though arrhythmias may occur secondary to hyperkalemia and hypocalcemia during acute myoglobinuric crises. Statistically speaking, classic CPT II deficiency is the most common cause for recurrent myoglobinuria in children and adults. However, a number of cases of severe infantile CPT II deficiency have been described which have included recurrent Reye-like syndrome, hepatomegaly with hypoketotic hypoglycemia and elevated liver aminotransferase, and cardiomegaly with cardiac arrhythmias and elevated serum creatine kinase, as well as evidence of lipid storage in heart, skeletal muscle, liver, and kidney. In these cases the activity of CPT II in cultured skin fibroblasts was<10% of controls in contrast to the 25% residual activity documented in classic CPT II deficiency. A third phenotype is the lethal neonatal form which may have multiple congenital anomalies including CNS malformations that include polymicrogyria, agenesis of the corpus callosum, and ventriculomegaly, seizures, cardiomegaly with hypertrophy, hepatomegaly and enlarged kidneys with renal cysts and neonatal death in which there are null mutations with<5% residual CPT II activity. More than 64 mutations have been described in the literature. Thus the clinical presentation of CPT II deficiency may depend, in part, on the degree of residual CPT II activity.
There is another plasma membrane fatty acid oxidation disorder which involves a defect in the active transport of long-chain free fatty acids across the plasma membrane. This has been described in two young boys who presented with acute liver failure with hyperammonemia, hyperbilirubinemia, coagulopathy and mild encephalopathy with no evidence of cardiac or skeletal myopathy, and who required liver transplantation. One boy had documented hypoketotic hypoglycemia. The uptake of oleic acid (C18:1) in skin fibroblasts from the patient was lower than in controls. The features which were atypical for an FAO defect included the absence of fatty liver with low concentrations of long-chain free fatty acids and elevated carnitine concentrations, due to defective transport of long chain free fatty acids.
There are many common features shared by the intramitochondrial β-oxidation defects (SCAD, MCAD, LCAD, VLCAD, ETF, ETF-CoQ, SCHAD, M/SCHAD, trifunctional protein/LCHAD, MKAT, 2,4,-dienoyl-CoA reductase, HMG-CoA synthase, and HMG-CoA lyase deficiencies), though there are also differentiating characteristics. It should be noted that many cases previously attributed to LCHAD deficiency are now known to be related to a defect in the trifunctional protein (TFP) which combines the activities of the long-chain enoyl-CoA hydratase, long-chain L-3-hydroxyacyl-CoA dehydrogenase, and long-chain 3-ketoacyl-CoA thiolase enzymes although isolated LCHAD deficiency appears to be more common than TFP deficiency. A number of previously diagnosed cases of LCAD deficiency are now believed to be attributable instead to VLCAD deficiency. Cardiac involvement (cardiomegaly, cardiomyopathy) is more commonly found in the long-chain defects (e.g. LCAD, VLCAD, trifunctional protein), but has also been documented in one case of SCHAD deficiency and one case of SCAD deficiency. Cardiomyopathy has been documented in 17 of 24 reported cases of TFP deficiency with presumed or proven marked LCHAD deficiency.
Chronic skeletal muscle weakness is seen in all of the intramitochondrial β-oxidation defects, but less commonly in MCAD and HMG-CoA lyase deficiency. A unique case of SCAD deficiency has been reported in a 13-year-old girl with progressive external ophthalmoplegia, ptosis, cardiomyopathy, and multicore myopathy. Additional cases of SCAD deficiency with multicore myopathy have now been identified. Recurrent myoglobinuria has been reported with LCAD, VLCAD, trifunctional protein/LCHAD, SCHAD, MCKAT, and MCAD deficiencies. In a long-term follow-up study of 13 patients with VLCAD deficiency, all patients exhibited exercise intolerance and recurrent myoglobinuria triggered by strenuous exercise, fasting, cold, or fever with a mean age at onset of 10 years. Muscle biopsies showed mild lipid accumulation in four patients. Perinatal onset of VLCAD deficiency has been reported in a male neonate with fetal distress, neonatal asphyxia, and transient hyper-creatine kinasemia (8400 IU/L) followed by repeated episodes of myoglobinuria one to two times/year during infancy and early childhood. Ohashi et al. showed that immuno-histochemistry is an effective and useful diagnostic method for the detection of VLCAD deficiency, which facilitated identification of 13 new Japanese patients with VLCAD deficiency. Recurrent myoglobinuria has been reported in TFP deficiency beginning at 7 months of age. An episode of myoglobinuria has also been described in an adult with MCAD deficiency.
All of the intramitochondrial enzyme defects demonstrate hepatic dysfunction at the time of acute catabolic decompensations; however, persistent dysfunction has only been documented in trifunctional protein/LCHAD and LCAD/VLCAD deficiencies . Of note, there is increasing evidence that a significant subgroup of women with acute fatty liver of pregnancy (AFLP) or hemolysis, elevated liver enzymes, and low platelets (HELLP) syndrome are heterozygous for LCHAD deficiency and carry affected LCHAD deficient fetuses with a common mutation (G1528C, E474Q) in one or both alleles. In AFLP syndrome, the mother may suffer fulminant liver failure and death. In both syndromes there is microvesicular fatty infiltration of the maternal liver. In addition to the recurrent episodes of myoglobinuria, TFP/LCHAD deficiency may present with progressive pigmentary retinopathy and motor-sensory neuropathy. Thus, individuals with the myoneuropathic variant of TFP/LCHAD deficiency may mimic the spinal muscular atrophy phenotype. There appear to be three major phenotypes of TFP/LCHAD deficiency with variable overlap; a hepatic form with Reye-like syndrome which is associated with AFLP, a neonatal cardiomyopathic form, and a milder myoneuropathic form. These phenotypes may correlate with specific genotypes and may be a reflection of the severity of the mutation and the presence of additional environmental and genetic factors.
Maternal complications in pregnancy are not limited to carriers of LCHAD mutations. Patients with deficiencies of CPT I, MCAD, and SCAD were also born to mothers who developed liver disease during their pregnancies. Therefore all babies born following pregnancies that are complicated by AFLP or HELLP syndrome should be considered at risk for having a defect in β-oxidation. A further complication of pregnancy includes placental floor infarction documented in the pregnancy of a fetus with LCHAD deficiency.
SCAD deficiency is a clinically heterogeneous disorder in which the clinical phenotype varies from fatal metabolic decompensations including intermittent metabolic acidosis and neonatal hyperammonemia to infantile onset lipid storage myopathy with failure to thrive, developmental delay, hypotonia, seizures, and hyperreflexia to more subtle later onset progressive myopathy including multicore myopathy and to entirely asymptomatic individuals. The characteristic metabolites of ethylmalonic and methylsuccinic acids in SCAD deficiency may also be due to the presence of one of two relatively common variants of SCAD (625G>A and 511C>T) which predispose to excessive ethylmalonic acid production and are also present in normal populations. These common variants may represent disease susceptibility variations when occurring in combination with other genetic and/or environmental factors, especially fever. They encode proteins with nearly normal enzymatic activity at physiological conditions in vitro ; however, SCAD enzyme function is impaired at increased temperature and the tendency to misfold increases under conditions of cellular stress. We have further demonstrated that vulnerability to oxidative stress may contribute to the pathophysiology of SCAD deficiency in patients homozygous for the common variant 625G>A, 625A/319T compound heterozygous patients, and patients homozygous for the pathogenic 319C>T and 1138C>T mutations. The c.319C>T ACADS gene mutation presents with clinical heterogeneity and appears to be a candidate founder mutation in the Ashkenazim. The challenge with SCAD deficiency is to elucidate whether ACADS gene variations are disease-associated, especially when combined with other genetic/cellular/environmental factors such as fever, which may act synergistically.
Multiple acyl-CoA dehydrogenase deficiency or glutaric acidemia type II is due to defects of the electron transfer flavoprotein (ETF) or ETF-ubiquinone oxidoreductase (ETF-QO) which are nuclear encoded proteins through which electrons from flavoprotein acyl-CoA dehydrogenases, dimethylglycine dehydrogenase, and sarcosine dehydrogenase enter the respiratory chain. These patients can be divided into three groups, each consistent within a family and have been designated as (1) neonatal onset with congenital anomalies, (2) neonatal onset without anomalies, and (3) mild or later-onset. The first two groups are sometimes said to have severe multiple acyl-CoA dehydrogenase deficiency (MADD) and the third group to have mild MADD. Neonatal onset patients with congenital anomalies are often premature, presenting within the first 24 to 48 hours of life with severe hypoglycemia, hypotonia, hepatomegaly, and metabolic acidosis and an odor, similar to sweaty feet. The infants may have enlarged kidneys and facial dysmorphism (high forehead, low-set ears, hypertelorism, hypoplastic mid face, etc.), rocker-bottom feet, muscular defects of the anterior abdominal wall, and anomalies of the external genitalia (hypospadias, chordee). Most of these patients die within the first week of life. In others, congenital anomalies are not noted, but renal cysts are found at necropsy. The infants without congenital anomalies usually develop hypotonia, tachypnea, hepatomegaly, hypoglycemia, metabolic acidosis, and a “sweaty foot” odor within the first few days of life, many in the first 24 hours. The few infants with this form who have survived beyond the first week of life due to prompt diagnosis and treatment, have died within a few months, usually with severe cardiomyopathy. A few other infants have been hypoglycemic in the newborn period and later developed typical Reye-like episodes and have survived longer.
Routine laboratory evaluation shows severe metabolic acidosis, mild to moderate hyperammonemia, and severe hypoglycemia without serum or urinary ketones. Serum transaminases may be elevated and prothrombin and partial thromoplastin times may be prolonged. Plasma lactate is usually elevated. There may be hypertrophic cardiomyopathy and renal cysts. Urinary organic acids show various combinations of short-chain volatile acids (e.g. isovaleric, isobutyric, 2-methylbutyric); glutaric, ethylmalonic, 3-hydroxyisovaleric, 2-hydroxyisoglutaric, 5-hydroxyhexaenoic, adipic, suberic, sebacic, and dodecanedioic acids; and isovalerylglycine, isobutyrylglycine, and 2-methylbutyrylglycine. Patients with neonatal onset may have generalized aminoacidemia and aminoaciduria. Plasma carnitine concentrations may be low or normal but acylcarnitine esters are increased in the urine.
Late onset glutaric aciduria type II (GA II) is extremely variable in age at presentation and course of the disease. One infant presented at 7 weeks of age with intermittent episodes of vomiting, hypoglycemia and acidosis, whereas another individual was asymptomatic during childhood and presented in adult life with episodic vomiting, hypoglycemia, hepatomegaly, and proximal myopathy. Some have had progressive lipid storage myopathy with carnitine deficiency. A 12-year-old girl presented with nausea, vomiting, and depression followed by progressive muscle weakness, elevated CK, and lipid storage myopathy which responded well to riboflavin, L-carnitine, and a high calorie, fat-reduced diet. For the riboflavin responsive-MAD deficiency, the challenge is to find the connection between variations in the ETFDH gene and the observed deficiency of a number of different mitochondrial dehydrogenases as well as deficiency of FAD and coenzyme Q10. Isolated myopathic coenzyme Q10 deficiency is caused by mutations in the ETF dehydrogenase gene and can be treated with CoQ10 and riboflavin supplementation. Patients present with exercise intolerance, fatigue, proximal myopathy, and high CK with lipid storage myopathy, subtle signs of mitochondrial myopathy, and severely decreased activities of respiratory chain complexes I and II+III, moderately decreased complex IV, and significantly decreased CoQ10 in muscle. Late-onset GA II and myopathic CoQ10 deficiency may be allelic diseases.
SCHAD deficiency has only been rarely reported. It is also now known as medium- and short-chain L-3-hydroxyacyl-CoA dehydrogenase (M/SCHAD) deficiency, given its broader chain length specificity. Furthermore, it has been argued that it should be called HAD deficiency to distinguish it from the SCHAD enzyme, also known as 17beta-hydroxysteroid dehydrogenase type 10, that is not a member of the HAD family but instead belongs to the short chain dehydrogenase/reductase superfamily that acts on a wide spectrum of substrates, including steroids, cholic acids, and fatty acids, with a preference for short chain methyl-branched acyl-CoAs. Three distinct clinical and biochemical phenotypes have been described. One girl presented with recurrent myoglobinuria, myopathy, cardiomyopathy, and hypoketotic hypoglycemic encephalopathy and died at 16 years of age. SCHAD activity was deficient in muscle but not in cultured skin fibroblasts, though these fibroblasts demonstrated marked microvesicular steatosis when cultured in a glucose-free medium to simulate fasting stress. Other tissues were not examined enzymatically. A second phenotype presented with ketotic hypoglycemia and SCHAD deficiency was described in isolated mitochondria from cultured skin fibroblasts but no other tissues were studied. A third group of patients presented with fatal infantile hepatic involvement and steatosis and SCHAD activity was deficient in liver but normal in muscle and fibroblasts. However, Western blot analysis of SCHAD revealed an identical truncated protein in both liver and muscle from one patient, suggesting that SCHAD is similar in liver and muscle and that the normal activity in muscle may be due to other enzymes with C4 activity. Autopsy findings revealed marked steatosis and a muscle pattern consistent with spinal muscular atrophy in one patient. Mutation analysis of the SCHAD gene in patients with SCHAD deficiency has been inconclusive, suggesting that other proteins in addition to M/SCHAD may be necessary for enzymatic activity and that these proteins may have a tissue-specific distribution. Apparent disease-causing mutations have been described in a patient with fulminant hepatic failure that required transplantation. Hyperinsulinism with hypoglycemia has also been reported in SCHAD deficiency. The pathophysiology of hyperinsulinism in M/SCHAD deficiency is not yet fully understood; however, it is becoming increasingly evident that it results from an interaction of the SCHAD protein with glutamate dehydrogenase.
Only one patient with 2,4-dienoyl-CoA reductase deficiency in muscle and liver has been reported, who presented with neonatal hypotonia, microcephaly, a small VSD, and dysmorphism, and who died at 4 months of age of respiratory acidosis with biventricular hypertrophy at autopsy. The clinical significance of the biochemical abnormalities therefore cannot be confirmed until additional patients have been identified. In addition, one patient with medium-chain 3-ketoacyl-coenzyme A thiolase deficiency has been described in a Japanese male neonate who died at 13 days of age after presenting at 2 days of age with vomiting, dehydration, metabolic acidosis, liver dysfunction, and myoglobinuria.
There are several disorders of ketone body production. Deficiency of 3-hydroxy 3-methylglutaryl-CoA lyase (HMG-CoA lyase), which is also active in the metabolism of leucine, presents with hypoketotic hypoglycemia with hyperammonemia and acidosis. Succinyl-coenzyme A:3-ketoacid coenzyme A transferase (SCOT) functions in conjunction with mitochondrial acetoacetyl-coenzyme A thiolase for ketone body utilization (ketolysis) in extra-hepatic tissues. SCOT deficiency presents as persistent ketonuria in the first 1–2 years of life, while acetoacetyl-coenzyme A thiolase deficiency presents with variable clinical symptoms and exaggerated ketoacidosis in response to minor physiological stresses. HMG-CoA synthetase deficiency has been reported in a child who presented with fasting hypoketotic hypoglycemia. Remaining challenges in mitochondrial FAO such as MCAD, riboflavin responsive MAD and SCAD deficiencies are summarized well by Gregersen et al.
For illustrative examples, see Case Examples 40.1–40.5 .
A 9-year-old girl was originally born to unrelated asymptomatic parents of East Indian and Caucasian heritage. Her sister presented with congenital hypotonia and muscle atrophy, and at 1 year of age was found to have progressive cardiomyopathy with severe mitral regurgitation. This sibling also had failure to thrive and progressive motor deterioration and died at 3.5 years of age, at which time fatty infiltration of the liver was noted at necropsy.
The index patient was born a footling breech. At 1 month of age, a cardiac murmur was noted. By 14 months, her gross motor milestones plateaued, and over the next 2 years, she developed slowly progressive weakness and recurrent respiratory infections. At 3 years of age, she suffered from congestive heart failure with moderate-to-severe mitral regurgitation. Marked left ventricular dilatation was present on echocardiography. The ECG demonstrated peaked T waves in the anterolateral precordial leads. She was started on digoxin and diuretics. At 4 years 3 months of age, she was found to have very low carnitine concentrations in serum and in muscle. The total muscle carnitine was less than 5% of control values. A muscle biopsy showed lipid storage, but β-oxidation enzymes were normal. Serum creatine kinase was mildly elevated. Serum glucose was normal, as were urinary organic acids. There was no abnormal dicarboxylic aciduria. Oral carnitine was instituted at 1 g three times daily. Within 1 week, appetite, affect, and cardiac function had dramatically improved. By 4 weeks, she had gained weight and had markedly increased exercise tolerance and the ECG showed inversion of the formerly peaked T waves. The predicted left ventricular end systolic and end diastolic dimensions markedly improved from 240% and 205% of normal, respectively, to 175% and 160% of normal. By 4 months, she had good exercise tolerance, and by 7 months of therapy, the predicted left ventricular end systolic and end diastolic dimensions had decreased to 150% and 130% of normal, respectively. At 5 years of age, she had regained gross motor milestones. Her digoxin and diuretics were discontinued by 6.5 years. At 9 years of age, she had an IQ of 140 and was a competitive swimmer at school. On examination, she had a mildly myopathic facies, mild hypotonia, and persistent, although improving, fine motor delay. Her electromyogram was normal. Motor nerve conduction studies revealed borderline abnormalities. Her serum carnitine concentrations were low and she continued to have decreased renal reabsorption of carnitine. Her father’s serum carnitine concentrations were normal and her mother’s were low normal.
Carnitine uptake studies in the cultured skin fibroblasts of the patient showed minimal carnitine uptake. At a carnitine concentration of 5 μmol/L (K m value), the mean rate of uptake was 2% of control values. Carnitine uptake studies in the cultured skin fibroblasts of both parents revealed normal K m values of 5.0 μmol/L (controls=5.5±0.58) but reduced maximal rates of carnitine uptake with a V max of 1.33 pmol/min per mg protein (controls=3.4±0.36), which was 40% of control values. The normal K m values for carnitine binding affinity and reduced V max values for carnitine uptake in the heterozygote parents suggested the presence of a reduced number of normally functioning carnitine transporters. Family history of a previously affected sibling, as well as demonstrated heterozygosity in both parents on carnitine uptake studies, supported the autosomal recessive pattern of inheritance.
This boy presented at 12 years of age with recurrent episodes of muscle stiffness and pain lasting a few hours to 24 hours at a time following periods of prolonged exercise lasting greater than one hour at a time. On entry into competitive sports at age 15 years, following 2 to 3 hours of uninterrupted exercise, he began experiencing severe episodes of muscle stiffness and pain in his legs and shoulder girdle with subsequent weakness and coca-colored urine (myoglobinuria), which would last from 24 to 48 hours at a time. His serum creatine kinase could be elevated to >20,000 IU/L (normal<250) and would return to normal in a few days to a few weeks in time, as would his motor power following a period of rest and carbohydrate ingestion. These episodes could also be exacerbated by fasting, cold exposure and infection. Carbohydrate loading prior to exertion would reduce the episodes of pigmenturia. During university exam times with fasting and stress, he also noted that he felt jittery and weak, particularly in the morning prior to breakfast and was immediately improved following carbohydrate ingestion. He had full recovery of power between episodes. He never complained of true muscle cramps and had no second wind phenomenon. With participation in university level basketball and volleyball tournaments, he suffered multiple episodes of pigmenturia in one year. He had no documented episodes of acute renal failure. His brother had an identical clinical presentation with the exception of a probable episode of myoglobinuria at 2 years of age in association with fever and infection. His sister was asymptomatic. Parents were asymptomatic and nonconsanguineous. On neurological examination, the proband was an athletic, muscular young man with full power and no evidence of neuropathy or pigmentary retinopathy. On investigation several months after an episode of myoglobinuria, he had a normal forearm ischemic lactate test, excluding a defect in glycolysis or glycogenolysis. Muscle biopsy of the biceps was essentially normal. There was no evidence of lipid or glycogen accumulation, mitochondrial abnormalities, or necrosis or regeneration. His EKG and echocardiogram were normal. Fibroblast acylcarnitine profile, following incubation with palmitate, showed a slight accumulation of long-chain acylcarnitines. Enzymatic assay in cultured skin fibroblasts revealed normal CPT I activity but a 20% residual activity for CPT II, consistent with mild CPT II deficiency. Following institution of fatty acid oxidation treatment strategies and preventative measures, he had complete cessation of his episodes of myoglobinuria.
Statistically speaking, the most common cause for recurrent myoglobinuria in children and in adults is CPT II deficiency followed by phosphorylase deficiency (type V glycogenosis or McArdle disease). Exercise intolerance may begin in childhood for both conditions. However, overt episodes of myoglobinuria are usually seen in adolescence. These two disorders can be distinguished by clinical history and biochemical testing. In phosphorylase deficiency, there is a history of early onset muscle cramps during the initial stages of high-intensity isometric exercise (e.g. first 10 minutes) or with less intense but sustained dynamic exercise because carbohydrate is then the major source of energy. If the individual continues to exercise at a milder, low-intensity pace, they will then experience a “second wind” phenomena which will allow them to continue to exercise because of hepatic glycogenolysis providing the muscle with glucose as well as a progressive switch-over in muscle metabolism from a primary dependence on carbohydrate to fatty acid utilization for ATP generation. In classic adult-onset CPT II deficiency, individuals will be able to do short-burst, high-intensity exercise for short periods of time. In contrast, they will most frequently complain of muscle stiffness and myalgia following mild to moderate prolonged exercise (e.g. >1 hour) which may occur several hours after the exercise and which may last for several hours or days if an episode of myoglobinuria is precipitated. In CPT II deficiency, episodes may also be precipitated by fasting, infection, cold exposure, and stress. There is no second wind phenomenon . In phosphorylase deficiency there is often a persistent mild elevation in serum creatine kinase between episodes of myoglobinuria and there may be mild fixed proximal greater than distal weakness in approximately one-third of typical cases more commonly seen in older patients. In classic CPT II deficiency, the CK and power typically return to normal between catabolic crises. The “forearm ischemic lactate test” shows a normal three- to five-fold increase in serum lactate in the first 3 minutes in CPT II deficiency and in normal controls. This is paralleled by a similar increase in ammonia. In individuals with a defect in glycolysis/glycogenolysis, there is an insufficient rise in lactate (less than two-fold) and there will be a compensatory increase in ammonia, which also serves to indicate sufficient effort on the part of the individual. On muscle histochemistry with periodic acid-Schiff staining, there may or may not be focal accumulations of glycogen in the subsarcolemmal regions and between myofibrils in phosphorylase deficiency. The phosphorylase stain will show no staining in muscle fibers, though smooth muscle in the walls of intramuscular vessels will stain normally. In classic CPT II deficiency, the biopsy is usually normal following full recovery from episodes of myoglobinuria. However, mild microvesicular steatosis may be seen on Oil-red-O staining, if the individual is stressed with prolonged exercise or fasting prior to the biopsy.
This affected boy was born at 3.4 kg after a full-term uncomplicated pregnancy to healthy parents who had two other living children and a past history of a stillborn child. The parents were first cousins. The infant was well until 3 months of age, at which time, following a 3-day history of fever, he was found to be lethargic with respiratory arrest. He suffered partial and generalized seizures which were attributed to severe hypoglycemia (0.2 mmol/L). He had mild hepatomegaly and cardiomegaly (cardiothoracic index 0.57). He suffered cardiac arrhythmias (PVCs, atrioventricular block, and ventricular tachycardia) a few hours following admission to an intensive care unit. Biochemical analysis showed a plasma glucose of 0.7 mmol/L (3.5–6 mmol/L); no plasma or urinary ketones; arterial pH of 7.42; blood lactate of 2.5 mmol/L (0.6–1.9 mmol/L); serum potassium of 6.5 mmol/L (3.5–5.5 mmol/L); aspartate aminotransferase of 167 IU/L (5–25 IU/L); alanine aminotransferase of 91 IU/L (5–25 IU/L); blood ammonia of 75 μmol/L (20–40 μmol/L); fibrinogen of 1 g/L (2–4 g/L); creatine kinase of 1140 IU/L (15–100 IU/L) with MM isozyme of 1100 IU/L (15–100 IU/L) and MB isozyme of 34 IU/L (0–11 IU/L); lactate dehydrogenase of 870 IU/L (138–276 IU/L) with a normal profile of isozymes; and total plasma carnitine of 14 μmol/L (28–71 μmol/L) with esterified/total carnitine ratio of 80% (<30%). The infant recovered after 6 days of symptomatic therapy. L-carnitine administration restored the plasma carnitine to normal, total carnitine of 45 μmol/L and free of 31.5 μmol/L. Withdrawal of carnitine resulted in a fall in plasma carnitine concentration to 16 μmol/L in 1 week. Urinary organic acids, after cessation of L-carnitine, were normal. The infant subsequently had normal growth and development with mild persistent hepatomegaly. At 17 months of age, the child died suddenly after an overnight fast. The oxidation of palmitate was decreased by ~90% in this child’s cultured lymphoblasts and skin fibroblasts. CPT I activity in cultured skin fibroblasts was normal in contrast to the CPT II activity, which was reduced by 90% of that of controls.
A 30-month old girl presented in coma following 24 hours of fever, persistent vomiting, and progressive lethargy. She had normal vital signs but was unresponsive to deep pain and had mild hepatomegaly. Pertinent laboratory values on arrival included a blood glucose of 4 mg/dL, ammonia of 190 μg/dL, SGOT of 34 IU/dL, and SGPT of 21 IU/dL. A small to moderate amount of ketones was found by urine dipstix and a right middle lobe pneumonia was seen on chest X-ray. Within minutes of administering 20 mL of 50% dextrose, she began to respond to commands. After 12 hours of intravenous dextrose infusion and ampicillin, she had recovered completely. Ten months earlier, this child had been treated for a similar but milder episode of vomiting and lethargy without hypoglycemia or hyperammonemia. Family history was notable for two siblings who had died from acute illnesses that had been diagnosed as Reye syndrome. The first, a boy, had presented at 15 months of age with a seizure following 18 hours of vomiting and increasing lethargy. On arrival in the emergency room, he suffered a cardiac arrest and could not be resuscitated. No blood was drawn; however, a postmortem CSF glucose was 2 mg/dL in the absence of pleocytosis or bacteria. Reye syndrome was diagnosed on the basis of fatty degeneration of the liver at necropsy. The second sibling, a 15-month-old girl, suffered vomiting, hypoglycemia, hypotension, and coma 24 hours after uneventful cardiac surgery for repair of atrial and ventricular septal defects and she died within hours of her deterioration. Autopsy revealed marked fatty infiltration of the liver and a diagnosis of Reye syndrome was made. Another 4-year-old brother was healthy. There was no other family history of similar deaths, hypoglycemia, metabolic disease or consanguinity.
Organic acid analysis in the initial urine specimen of the index case revealed large amounts of saturated and unsaturated dicarboxylic acids, primarily adipic, suberic, and sebacic acids, but comparatively low levels of β-hydroxybutyrate and acetoacetate. Total serum carnitine concentration was decreased at 17 μmol/L (normal 40–60 μmol/L). Medium-chain acyl-CoA dehydrogenase deficiency was confirmed by enzyme assay of the patient’s mononuclear cells. Following diagnosis, the patient had two additional episodes of lethargy and vomiting with viral syndromes, each of which was treated with and responded quickly to intravenous 10% dextrose. Growth and development remained normal.
This 16-year-old-boy was born to asymptomatic first-cousin parents following a normal pregnancy and delivery. Gross motor milestones were delayed. At age 20 months, he suffered the first of many episodes of profound weakness precipitated by fever, vomiting, or dehydration. His subsequent clinical course was characterized by a slowly progressive limb-girdle myopathy with mild facial weakness and a symmetrical peripheral sensorimotor axonopathy with secondary demyelination. In addition, he suffered recurrent episodes of myoglobinuria with elevations in serum creatine kinase between 20,000 and 50,000 IU/L (up to 5 times per year). These episodes were precipitated by prolonged exertion, infection, cold exposure, fasting, and/or stress. They were intermittently accompanied by somnolence which responded promptly to intravenous glucose administration. Serum total carnitine concentration was 39 μmol/L (35–83 μmol/L) with a free carnitine of 19 μmol/L (24–63 μmol/L) and increased esterified fraction of 50%. Muscle biopsy revealed a mild lipid-accumulative myopathy with intramitochondrial lipid on electron microscopy. Sural nerve biopsy revealed axonal degeneration. During an acute catabolic episode, serum acylcarnitine assay revealed an elevation in long-chain acylcarnitines (C14–18) with 3-hydroxy species. Urinary organic acids revealed a moderate dicarboxylic aciduria with significant 3-hydroxydicarboxylic aciduria. The L-3-hydroxyacyl-CoA dehydrogenase activity measured with the long-chain substrate (C16) in cultured skin fibroblasts and biopsied muscle was significantly reduced. With the introduction of a frequent feeding, high-carbohydrate, low-fat diet and preventive fatty acid oxidation measures at age 7.5 yr, there was a marked reduction in the frequency of myoglobinuric episodes. However, no improvement in power or endurance occurred, and these continued to deteriorate. A trial of prednisone therapy resulted in a significant improvement in his limb-girdle myopathy, which has been sustained over 12 years, as well as a transient improvement in his peripheral neuropathy. The myoglobinuric episodes were markedly reduced.
Differentiating Laboratory Features
The site of biochemical defect may be suggested by the chain-length specificity and the species type of the fatty acid intermediates detected in the serum or urine of affected children. These intermediates require specialized biomedical technology which is generally not available in standard hospital clinical chemistry laboratories and may therefore require referral to specialized metabolic laboratories. Particularly useful are the assessment of plasma total and free carnitine, serum acylcarnitines, urine acylcarnitines, and urine acylglycines and organic acids. It should be emphasized that these intermediates may be absent at times when the child is metabolically stable and receiving adequate supplies of glucose, thereby eliminating the stress on the FAO pathway. They are therefore best detected in the serum and urine of children during acute catabolic crises or during times of fasting. The specific intermediates that are characteristic for the individual known defects are presented in Table 40.2 .
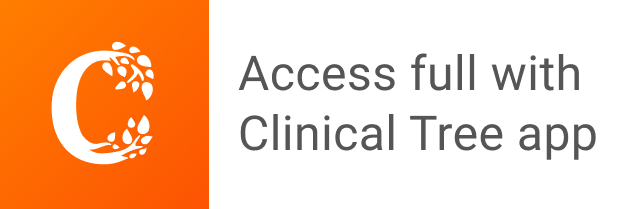