Magnetic Resonance Imaging of Acute Spinal Trauma
Adam E. Flanders
Prior to the development of magnetic resonance imaging (MRI), the extent of associated soft tissue injury to the intervertebral discs, ligaments, and spinal cord was determined primarily by inference from known biomechanical principles rather than by direct imaging of the affected tissues (1, 2). Consequently, many of the established therapies for spinal cord injuries (SCI) are based on radiologic classifications of osseous injury to the spinal column. These traditional therapeutic interventions for SCI are directed primarily by radiographic findings such as re-establishment of normal anatomic alignment of the spinal canal and removal of bone fragments. Current management of SCI, however, has become more directed toward the correction of the associated soft tissue and spinal cord damage (3, 4, 5 and 6), and MRI has become increasingly important in the diagnostic evaluation of spinal injuries.
The greatest impact that MRI has made in the evaluation of SCI has been in assessment of the intracanalicular and paraspinal soft tissues (3, 4 and 5—7, 8, 9, 10, 11, 12, 13, 14 and 15). The integrity of the intervertebral discs and ligamentous complexes can be routinely evaluated with MRI. In addition, MRI permits direct visualization of the morphology of the injured cord parenchyma and the relationship of the surrounding structures to the spinal cord (2, 16). No other imaging modality has been able to faithfully reproduce the internal architecture of the spinal cord, and it is this particular feature of MRI that promises to have the greatest impact on the management of the SCI patient in the future.
Although MRI is a powerful diagnostic tool, it has not supplanted conventional radiologic imaging in the initial evaluation of SCI (16). The conventional diagnostic algorithm of plain radiography supplemented by computed
tomography (CT) is the most appropriate and cost-effective method to evaluate most cases of spinal trauma (7, 10, 16, 17 and 18). MRI has replaced myelography and CT myelography (CTM), however, as the primary imaging option available to assess for residual soft tissue compression of the spinal cord (10, 12, 14, 19, 20) due to factors such as acute disc herniations and epidural hematomas. Identification of residual compression of the spinal cord has significant implications in regard to timing of subsequent surgery and the type of surgical approach that is required (8, 12, 20). MRI is also an essential diagnostic modality in cases of SCI without radiographic abnormality (SCIWORA) (11, 17, 20, 21, 22, 23, 24 and 25). An MRI examination in the acute period is warranted in any patient who has a persistent neurologic deficit after spinal trauma (10, 11, 17).
tomography (CT) is the most appropriate and cost-effective method to evaluate most cases of spinal trauma (7, 10, 16, 17 and 18). MRI has replaced myelography and CT myelography (CTM), however, as the primary imaging option available to assess for residual soft tissue compression of the spinal cord (10, 12, 14, 19, 20) due to factors such as acute disc herniations and epidural hematomas. Identification of residual compression of the spinal cord has significant implications in regard to timing of subsequent surgery and the type of surgical approach that is required (8, 12, 20). MRI is also an essential diagnostic modality in cases of SCI without radiographic abnormality (SCIWORA) (11, 17, 20, 21, 22, 23, 24 and 25). An MRI examination in the acute period is warranted in any patient who has a persistent neurologic deficit after spinal trauma (10, 11, 17).
The focus of this chapter is the application of MRI in the evaluation of injuries of the spinal axis and spinal cord. For a comprehensive reference of the radiology of spine trauma, the reader is encouraged to pursue the reviews found in Chapters 5, 6, and 7.
Demographics of Spinal Cord Injury
SCI is a significant cause of disability in the United States. Although the number of individuals who sustain paralysis yearly is substantially less than the number of people who sustain moderate to severe traumatic brain injury (TBI) (11,000 SCI per year versus 70,000 to 90,000 TBI per year), the financial costs to society for SCI are significant. Since most patients survive the acute SCI, there are approximately 225,000 to 288,000 SCI patients with partial or complete paralysis currently being cared for in the United States. The total lifetime costs for medical treatment and rehabilitation range from $200,000 to $800,000 per affected individual. The lifetime direct costs may exceed $2.8 million, for a high tetraplegic patient injured at age 25 (26, 27). Nearly 55% of all SCI occur in young adults between the ages of 16 to 30 years. Most SCI victims are white males (81.7%). The etiologies of SCI are vehicular (37.4%), acts of violence (25.9%), falls (21.5%), and sports injuries (7.1%) (27). Because SCI primarily affects employed (60.5%) young adults, there is a tremendous financial loss to society in terms of overall lifetime productivity.
Injuries to the spinal axis can be subdivided into spinal injuries (damage to the spinal axis without neurologic injury) and SCI (damage to the spinal cord with or without spinal axis abnormality). An accurate estimate of the total number of SCI is difficult to define because patients who expire in the field from a fatal SCI (i.e., high cervical cord) or from related injuries (e.g., cerebral trauma) are not included in the national statistics. Tetraplegia (quadriplegia) is defined as an injury to one of the eight cervical segments of the spinal cord with paralysis of all four limbs. Paraplegia usually results from injury to the thoracic, lumbar, or sacral segments of the spinal cord with dysfunction of both legs. A neurologically complete lesion is one in which there is no motor or sensory function three segments below the neurologic level of injury. Of those spinal cord-injured persons who survive to reach a medical facility, the most frequent neurologic deficit is incomplete tetraplegia (29.5%), followed by complete paraplegia (27.9%), incomplete paraplegia (21.3%), and complete tetraplegia (18.5%) (27). Less than 1% of SCI patients recover completely during the initial hospitalization.
Magnetic Resonance Imaging Techniques
Imaging Considerations
The spinal cord-injured patient requires special consideration before MRI with regard to patient transfer, life support, monitoring of vital signs, fixation devices, choices of surface coils, and pulse sequences. The potential risks from transporting a medically and neurologically unstable patient must be carefully weighed against the potential benefits derived from the diagnostic information provided by MRI. Most SCI patients can be accommodated with minimal risk to the patient as long as appropriate precautions are adhered to. As with all critical care patients, myriad life-sustaining devices may need to accompany the SCI patient to the MRI suite. Many of these devices are incompatible with the MRI environment. Conventional ventilatory equipment is safe to use only in the fringe field of ultralow field strength units. For mid- and high-field strength MRI units, several manufacturers offer MRI-compatible ventilators. The unit itself is operated remotely, and the ventilator controls remain inside the control room. Similarly, MRI-compatible monitors are now available that can relay heart rate, respiration, blood pressure, and oxygenation information directly into the MRI control area. Indwelling central venous catheters with thermocouples and conventional intravenous medication pumps are prohibited in the MRI environment.
External spinal fixation devices warrant special attention because, if used improperly in the MRI suite, they can pose a significant safety hazard to both the patient and personnel (28). Moreover, fixation devices that are composed of ferromagnetic alloys may destroy the static magnetic field close to the region of interest, resulting in image degradation.
Most patients will arrive for an MRI examination following closed reduction of the spinal injury and fixation with the appropriate external spinal stabilization device (Fig. 8-1). Occasionally, traction will be applied using cranial tongs and a system of pulleys and weights (Fig. 8-1C). Although it is possible to maintain traction on the scanning
table, it is not advisable to do so for several reasons: (a) it is cumbersome to attach the weights and pulleys to the scanning table, (b) the traction device may interfere with table motion, and (c) conventional traction weights (“sandbags”) contain metallic pellets that pose a significant projectile hazard to patient and personnel (Fig. 8-2) (28). Only MRI-compatible weights are permitted inside the scanning area (29).
table, it is not advisable to do so for several reasons: (a) it is cumbersome to attach the weights and pulleys to the scanning table, (b) the traction device may interfere with table motion, and (c) conventional traction weights (“sandbags”) contain metallic pellets that pose a significant projectile hazard to patient and personnel (Fig. 8-2) (28). Only MRI-compatible weights are permitted inside the scanning area (29).
Patients with cervical spine injuries are usually stabilized with a fiberglass cervical collar or, in more severe injuries, a halo and halo vest are used (30). For thoracic and lumbar injuries, the patient may be transported on a rigid spine board, a body cast, or in traction. MRI-compatible halo vests are often composed of a graphite composite, titanium, aluminum, and plastic, and are devoid of stainless steel components (31, 32). If the fixation pins used for femoral traction are ferrous, they usually do not interfere to any noticeable degree with the images of the spine, although tissue heating can occur at the contact points with skin.
Only properly trained personnel should perform transfer of a patient with spine instability to and from the scanning table. Patient motion should be minimized, as there is potential risk for further neurologic deterioration from moving of a patient with an unstable spine.
Patient motion (voluntary or involuntary) can also be detrimental to the quality of any MRI study. Even a patient with acute tetraplegia can seriously degrade a cervical MRI examination either by movement of the head and neck or from irregular ventilation. Sedation may be necessary to complete an examination.
Choice of surface coil is determined by the location(s) of injury, access to the area of interest, and the types of coils available. The proximity of the surface coil to the area of interest is a key factor in determining image quality. Temporary removal of a cervical collar, for example, will permit the use of specially designed quadrature or anterior/posterior neck coils. Wherever possible, a dedicated spine-phased array coil system should be used to maximize coverage and optimize MRI signal.
When the neck is fixed in a halo vest (Fig. 8-1A,B), use of a phased-array coil system can be problematic as the distance between the coil surface is increased, which can diminish the returned signal strength. Instead, a pair of 5-inch circular surface coils closely applied to the back of the neck can be used effectively. A multicoil phased-array system can be accommodated in most thoracic and lumbar evaluations. Alternatively, the patient can be placed over a sliding surface coil tray, which permits repositioning of the coil without moving the patient. A conventional 5-inch by 11-inch “license plate” surface coil provides adequate coverage for sagittal fields of view up to 32 cm.
When the neck is fixed in a halo vest (Fig. 8-1A,B), use of a phased-array coil system can be problematic as the distance between the coil surface is increased, which can diminish the returned signal strength. Instead, a pair of 5-inch circular surface coils closely applied to the back of the neck can be used effectively. A multicoil phased-array system can be accommodated in most thoracic and lumbar evaluations. Alternatively, the patient can be placed over a sliding surface coil tray, which permits repositioning of the coil without moving the patient. A conventional 5-inch by 11-inch “license plate” surface coil provides adequate coverage for sagittal fields of view up to 32 cm.
MRI evaluation of the spine following penetrating trauma requires special consideration for two reasons: (a) retained metallic fragments within the spinal canal may be regarded as a safety hazard to the patient, and (b) depending on their composition, these fragments can produce significant local image degradation (Fig. 8-3). Most firearm projectiles are nonferrous and therefore will not move in the static magnetic field (33). A ferrous fragment in the spinal canal could theoretically migrate, dislodge, or produce thermal injury when exposed to the strong static magnetic field and radiofrequency energy, potentially producing further neurologic injury (34). Bullets encased in steel, copper, or copper-nickel exhibit significant deflection force in a high-field MRI unit (34). Although we are not aware of any reports of ascending paralysis from retained bullet fragments in the spinal canal, any MRI examination following penetrating trauma to the spine should be performed at the discretion of the radiologist after review of radiographs or CT of the area of interest. Additional consideration should be given to the length of time the fragment has been embedded in the tissue as the potential risk of movement is diminished in mature scar tissue. If there are sufficient safety concerns, then myelography or CTM should be used.
Imaging Methods
At a minimum, evaluation of the injured spine should be performed both in the axial and sagittal planes using a
combination of pulse sequences. At a minimum, T1- and T2-weighted information are both necessary to completely assess the spinal axis and the spinal cord. Additional sequences are performed as needed, depending on the portion of the spine that is injured, the degree of injury, and patient tolerance. Conventional fast spin-echo (FSE) or turbo spin-echo (TSE) and gradient-echo pulse sequences are used most often (Table 8-1).
combination of pulse sequences. At a minimum, T1- and T2-weighted information are both necessary to completely assess the spinal axis and the spinal cord. Additional sequences are performed as needed, depending on the portion of the spine that is injured, the degree of injury, and patient tolerance. Conventional fast spin-echo (FSE) or turbo spin-echo (TSE) and gradient-echo pulse sequences are used most often (Table 8-1).
Table 8-1 Proposed Trauma MR Scan Protocol | |||||||||||||||||||||||||||
---|---|---|---|---|---|---|---|---|---|---|---|---|---|---|---|---|---|---|---|---|---|---|---|---|---|---|---|
|
An inherent property of the FSE pulse sequences is that the images exhibit less magnetic susceptibility artifact
as compared to conventional spin-echo and gradient-echo images (35). Although this property may seem theoretically disadvantageous when searching for small areas of acute spinal cord hemorrhage, FSE images have been shown to be comparably sensitive to conventional spin-echo images for detecting intramedullary hemorrhage (36). The decrease in magnetic susceptibility with FSE may be advantageous when imaging postoperative spines with instrumentation that otherwise would be obscured by artifacts (Fig. 8-4) (35). Use of hybrid techniques such as GRASE (gradient spin-echo) may provide improved visualization of intramedullary hemorrhage in SCI over FSE images; however, the increase in artifacts and noise may prohibit its routine use in this application (Fig. 8-5) (37). Manually increasing receiver bandwidth (RBW) decreases the read-out period, which has the added benefit of diminishing susceptibility effects (Fig. 8-4).
as compared to conventional spin-echo and gradient-echo images (35). Although this property may seem theoretically disadvantageous when searching for small areas of acute spinal cord hemorrhage, FSE images have been shown to be comparably sensitive to conventional spin-echo images for detecting intramedullary hemorrhage (36). The decrease in magnetic susceptibility with FSE may be advantageous when imaging postoperative spines with instrumentation that otherwise would be obscured by artifacts (Fig. 8-4) (35). Use of hybrid techniques such as GRASE (gradient spin-echo) may provide improved visualization of intramedullary hemorrhage in SCI over FSE images; however, the increase in artifacts and noise may prohibit its routine use in this application (Fig. 8-5) (37). Manually increasing receiver bandwidth (RBW) decreases the read-out period, which has the added benefit of diminishing susceptibility effects (Fig. 8-4).
![]() FIGURE 8-5. GRASE (gradient spin echo) sagittal image of a cervical injury. Note the increased amount of background noise associated with this technique. |
Imaging of the cervical, thoracic, or lumbar regions begins with a low-resolution gradient-echo localizer in the coronal plane. This image(s) can be obtained in less than 1 minute and can be used subsequently to prescribe the sagittal sequences. A maximum of nine to 12 sagittal images are usually required to interrogate the spine to include the lateral elements. Sagittal images should be no more than 3 to 4 mm thick with a 0- to 1-mm slice gap. The field of view of the area of interest is adequate at 22 to 24 cm. In the thoracic spine, a large sagittal localizer is also needed (48 cm field-of-view) for accurate labeling of the involved levels.
Modern MRI equipment has the capacity to rapidly image multiple regions of the spine simultaneously without repositioning the patient. With the availability of combined head and spine array surface coils and moving MRI tables, the entire spine can be imaged without repositioning the patient. This “survey” method may be useful when multiple levels of the spinal axis need to be rapidly interrogated at one time (Fig. 8-6).
The prescribed spatial resolution depends on the inherent limitations of the MR scanner, the type of sequence used and acquisition time limitations. T2-weighted information is obtained using a single FSE acquisition using a split echo train, resulting in an intermediate and T2-weighted image. Alternatively, two separate FSE acquisitions can be used with different echo train lengths. To produce a short effective echo time (TEff) image (intermediate weighted) an echo train of four with two excitations is suggested, whereas for the long TEff image an echo train of eight with four excitations is recommended. At a minimum, 256 steps are prescribed in both the frequency (xres) and phase (yres) axes. Unlike spin echo sequences, fat-containing structures remain bright on FSE/TSE images due to the effects of J-coupling. Therefore, fat suppression must be employed on the long repetition time (TR) sequences to improve visualization of edema in the posterior ligamentous complexes. Typically, a spectrally selective fat-saturation pulse is applied that is tuned to the resonance frequency of lipid on a T2-weighted image. Alternatively, a short tau inversion recovery (STIR) sequence can be employed to produce effective suppression of lipid signal in the soft tissues, thereby improving the conspicuity of edema. Increased signal-to-noise and shorter acquisition times can be achieved by applying driven equilibrium pulses (38). Fat saturation techniques can have a deleterious effect on image quality when ferromagnetic hardware is present (Fig. 8-4). When available, parallel imaging methods can be advantageous in spinal imaging by reducing imaging time, reducing blurring in FSE/TSE sequences, and by helping to reduce motion artifacts (39). The phase-encoding axis is oriented parallel to the spine so that phase ghosting is not propagated across the spinal canal. A form of gradient moment nulling (GMN) should also be employed in the cervical and thoracic regions to compensate for cerebrospinal fluid (CSF) flow artifacts. Cardiac gating is another option for correcting cerebrospinal flow artifacts on T2-weighted sequences. Anteriorly placed saturation pulses are helpful in reducing artifacts produced by swallowing, breathing, and cardiac motion. Respiratory compensation may be of benefit when imaging the thoracic and lumbar regions. Resolution can be maintained with reduced imaging times by implementing options that vary the number of phasing encoding steps and field of view.
Cross-sectional information on MRI is essential, especially when assessing the cervical and thoracic spinal cord. The choice of axial pulse sequence varies, depending on the part of the spine being evaluated, extent of injury, type of tissue contrast required, personal preferences, and time constraints. Usually, axial images that provide hyperintense CSF are preferred and are obtained using gradient-echo (GE) or FSE pulse sequences. In the cervical spine, a myelographic-like image is produced using an axial three-dimensional Fourier transform (3D FT) GE pulse sequence to obtain 28 or 64 contiguous images, at 1.5 mm thickness. Technical parameters include 5-degree flip angle, minimum TR/TE, 256 by 192 matrix and two excitations. The TE should be <15 msec in order to minimize unwanted susceptibility effects that might exaggerate bony stenoses (40). To maximize detection of acute intramedullary hemorrhage, at least one GE sequence should be performed. High-resolution cross-sectional imaging of the spinal cord can be performed using FSE techniques in the study of the cervical and thoracic spine.
As a supplement to the cervical examination, a survey of the extracranial vasculature is useful to detect posttraumatic occlusion or dissection of the carotid and vertebral arteries. This may be achieved with routine two-dimensional (2D) time-of-flight (TOF) magnetic resonance angiography (MRA), 3D TOF MRA, or a contrast-enhanced MRA (CEMRA) using ellipticocentric reordering of k-space. An axial cross-sectional evaluation of the neck using a T1-weighted “black-blood” technique (employing superior/inferior spatial and fat saturation) is helpful to identify subtle arterial dissections of the extracranial vasculature.
Although there are reported cases in which gadolinium was useful in the evaluation of acute SCI, the justification for routine use is unsubstantiated (41, 42, 43 and 44). In humans, some degree of enhancement of the posttraumatic spinal cord lesion has been reported at 1 to 14 weeks after injury (43, 44). The enhancement is postulated to represent breakdown of the blood-brain barrier in the acute phase and reparative granulation tissue late after injury (43, 44). In our experience, contrast agents have no clinical utility in the routine MRI evaluation of acute spinal trauma.
Characterization of Spinal Injury Using Magnetic Resonance Imaging
Although the biomechanics and types of injuries to the spine vary by location, the observed soft tissue and osseous changes to the spinal axis and spinal cord on MRI are relatively similar. Any interpretation of an MRI examination performed for spinal injury should include a discussion of the integrity of the intervertebral discs, vertebral bodies, vertebral alignment, ligaments, and neural elements. Specifically, the types of changes that are observed with MRI in SCI can be grouped into osseous injuries, ligamentous and joint disruption, intervertebral disc injury, fluid collections, vascular injury, and SCI. The force of injury is often dissipated primarily at one level in the spine; therefore, injury to all of the tissues (e.g., bone, ligament, disc, and spinal cord) is usually anticipated at one to two isolated levels.
Identification of injury to one tissue type should prompt the observer to scrutinize the same level for injuries to other tissues.
Identification of injury to one tissue type should prompt the observer to scrutinize the same level for injuries to other tissues.
Most of the diagnostic information in spinal cord injury is derived from the sagittal images. Axial images serve as a supplement (2). Sagittal T1-weighted images offer an excellent anatomic overview. Disc herniations, epidural fluid collections, subluxations, vertebral body fractures, cord swelling, and cord compression are also visualized (18). The fat-suppressed sagittal T2-weighted images are usually relied upon to depict most of the soft tissue abnormalities including spinal cord edema and hemorrhage, ligamentous injury, disc herniation, and epidural fluid collections (13). Axial and sagittal GE images aid in the identification of acute spinal cord hemorrhage, disc herniations, and fractures. MRI has not been successful in reliably demonstrating traumatic nerve root avulsions. Occasionally, posttraumatic root pouch cysts are identified. High-resolution T2-weighted cross-sectional images may reveal traumatized rootlets (Fig. 8-7). CT with intrathecal contrast remains the diagnostic method of choice for demonstrating the characteristic empty nerve root sheath and the periradicular cavities (45, 46).
Osseous Injury
Currently, MRI does not offer any advantage over plain radiography or high-resolution multidetector CT (MDCT) in the evaluation of associated osseous injuries following spinal trauma (8, 16, 18, 24). Moreover, even when MRI is available, it should only be performed after appropriate radiographic evaluation of the osseous injury.
The overall incidence of cervical spine injury from a multi-institutional survey of 615 US trauma centers was estimated to be 4.3%. The incidence of cervical spine injury without SCI was 3.0% and the incidence of cervical SCI without fracture was 0.7% (47).
The traumatic osseous changes to the spinal axis on MRI are divided into subluxations, fracture deformities, and compressive injuries. Relative loss of alignment at a specific level of the spinal axis is readily depicted on a midsagittal MRI image. The sensitivity of MRI in detecting anterior subluxation is probably better than conventional radiography or CT because the morphology of the thecal sac is also demonstrated and because portions of the spine obscured on plain radiography (e.g., cervical-thoracic junction) are clearly identified on MRI (16).
Nondisplaced fracture lines through the vertebral bodies and posterior elements are poorly demonstrated on MRI. The fracture line is sometimes visible on GE images as a thin hyperintense band that traverses the vertebral body. Depending on the mode of injury, this band may be oriented vertically, horizontally, or obliquely (Figs. 8-8, 8-9, 8-10, 8-11, 8-12, 8-13 and 8-14) (2, 10, 12, 16). A fracture line that extends through the cortex may interrupt the continuity of the characteristic hypointense peripheral margin of cortical bone (2, 10). It may be difficult to distinguish cortical bone fragments
from ligament on MRI because both structures exhibit low signal intensity on all pulse sequences (48). Displaced fractures produce concomitant deformity of the involved vertebral body and, if directed posteriorly, compress on the thecal sac. The latter is readily apparent on sagittal images (2). Although identification of a vertebral body or posterior element fracture is not predictive of a neurologic deficit, burst fractures do have a high propensity for associated neurologic deficit (8, 16).
from ligament on MRI because both structures exhibit low signal intensity on all pulse sequences (48). Displaced fractures produce concomitant deformity of the involved vertebral body and, if directed posteriorly, compress on the thecal sac. The latter is readily apparent on sagittal images (2). Although identification of a vertebral body or posterior element fracture is not predictive of a neurologic deficit, burst fractures do have a high propensity for associated neurologic deficit (8, 16).
MRI is notoriously insensitive to all types of fractures involving the posterior elements (Fig. 8-8) (5, 16, 24, 49, 50 and 51). This decreased sensitivity is attributed to the smaller size, the complex geometry, and the lower proportion of medullary space of the posterior elements relative to the vertebral bodies. These characteristics are particularly true in the cervical spine (2). Comparison of axial CT and axial GE MRI images shows that MRI has low sensitivity and moderate specificity for posterior element fractures (8, 49). Moreover, fractures of C1 and C2 are also extraordinarily difficult to demonstrate on MRI (Fig. 8-15). In an evaluation of 32 patients with cervical spine fractures, MRI was found to have a sensitivity of 36.7% in the detection of anterior column fractures and 11.5% sensitivity for posterior element fractures in comparison to CT (51). The improved sensitivity to anterior column injury was attributed partly to marrow edema, which served as an indicator of deformity.
MRI is unique, however, in its ability to demonstrate compressive injury to the marrow elements even without evidence of fracture deformity or cortical failure. Compressive injury is manifested by hypointensity of the marrow space within the involved vertebral body on the short TR images and relative hyperintensity on the long TR images (Figs. 8-16, 8-17) (2, 8, 9, 12, 52). These signal alterations presumably are the result of microfractures within the medullary bone and resultant hemorrhage. As these signal changes are transient, they can be used as a secondary indicator of an acute osseous injury.
Ligamentous and Joint Disruption
MRI is the only imaging modality available that directly visualizes changes to the ligaments as a result of trauma. The ligamentous structures that are readily identified on routine sagittal MRI of the spine include the anterior longitudinal ligament (ALL), posterior longitudinal ligament (PLL), ligamentum flava (LF), and interspinous ligaments (ISP) (Fig. 8-18). They are relatively avascular structures composed primarily of strong fibroelastic tissue with very short T2 relaxation properties. Therefore, ligaments appear relatively hypointense to other structures on all
MRI pulse sequences. When overstretched or ruptured, a gap in the ligament may be identified and the surrounding tissues may increase in signal intensity on T2-weighted or GE images because of an increase in free water content from extracellular fluid or adjacent hemorrhage (9, 10, 16). Because of the similarity in imaging characteristics, distinction between a ligament fragment and cortical bone fragment may prove difficult on MRI (16, 48).
MRI pulse sequences. When overstretched or ruptured, a gap in the ligament may be identified and the surrounding tissues may increase in signal intensity on T2-weighted or GE images because of an increase in free water content from extracellular fluid or adjacent hemorrhage (9, 10, 16). Because of the similarity in imaging characteristics, distinction between a ligament fragment and cortical bone fragment may prove difficult on MRI (16, 48).
The longitudinal ligaments are solitary, continuous strips of fibroelastic tissue that extend from the skull base to the sacrum. They function to maintain vertebral body alignment and provide elasticity during flexion, extension,
and rotation. Failure of either ligament at any spinal level is indicative of spinal instability (Figs. 8-19, 8-20, 8-21, 8-22, 8-23, 8-24, 8-25, 8-26, 8-27, 8-28, 8-29, 8-30, 8-31 and 8-32). On MRI the ALL is a thin, continuous band of low signal intensity that lies ventral to the anterior cortical surface of the vertebral bodies (53, 54, 55). The ALL is a critical component of the anterior column, which includes the anterior one half of the vertebral body and annulus fibrosis. Normally, the ALL may be indiscernible from the cortex or the outer annulus of the intervertebral disc; however, when elevated by fluid, disc, or bone, it may be more apparent (Figs. 8-20, 8-21 and 8-22) (55). Portions of the ligament merge with Sharpey fibers at the vertebral endplate and
with the outer annular fibers. The ALL may rupture as the result of hyperextension injury (Figs. 8-27, 8-28) (17, 53, 56, 57, 58). This is seen on all pulse sequences as a focal discontinuity of the hypointense band that is adherent to the ventral aspect of the vertebral bodies (Figs. 8-22, 8-23 and 8-24). This finding may be associated with an avulsion of the vertebral endplate (Figs. 8-22, 8-23 and 8-24) or hemorrhage in the prevertebral musculature (17, 53, 57, 58). The accumulation of hemorrhage and fluid in the prevertebral space is seen on T2-weighted or GE sequences as a crescent-shaped mass of high signal intensity centered over the segment of injured ligament (Figs. 8-21, 8-22, 8-23, 8-24 and 8-25) (14, 24, 53).
and rotation. Failure of either ligament at any spinal level is indicative of spinal instability (Figs. 8-19, 8-20, 8-21, 8-22, 8-23, 8-24, 8-25, 8-26, 8-27, 8-28, 8-29, 8-30, 8-31 and 8-32). On MRI the ALL is a thin, continuous band of low signal intensity that lies ventral to the anterior cortical surface of the vertebral bodies (53, 54, 55). The ALL is a critical component of the anterior column, which includes the anterior one half of the vertebral body and annulus fibrosis. Normally, the ALL may be indiscernible from the cortex or the outer annulus of the intervertebral disc; however, when elevated by fluid, disc, or bone, it may be more apparent (Figs. 8-20, 8-21 and 8-22) (55). Portions of the ligament merge with Sharpey fibers at the vertebral endplate and
with the outer annular fibers. The ALL may rupture as the result of hyperextension injury (Figs. 8-27, 8-28) (17, 53, 56, 57, 58). This is seen on all pulse sequences as a focal discontinuity of the hypointense band that is adherent to the ventral aspect of the vertebral bodies (Figs. 8-22, 8-23 and 8-24). This finding may be associated with an avulsion of the vertebral endplate (Figs. 8-22, 8-23 and 8-24) or hemorrhage in the prevertebral musculature (17, 53, 57, 58). The accumulation of hemorrhage and fluid in the prevertebral space is seen on T2-weighted or GE sequences as a crescent-shaped mass of high signal intensity centered over the segment of injured ligament (Figs. 8-21, 8-22, 8-23, 8-24 and 8-25) (14, 24, 53).
![]() FIGURE 8-21. Flexion-rotation injury at C4-5. A: Sagittal FSE T2-weighted image. The body of C4 is subluxed relative to C5. A moderate-sized disc herniation (curved black arrow) has impacted on the swollen and edematous spinal cord. No blood products are identified in the spinal cord. Note the separation of the PLL from the midportion of the C4 body (long black arrow). The ventral dura margin is represented by a thin hypointense line (small black arrows). There is associated disruption of the ligamentum flavum (black arrow) and the interspinous ligaments (asterisk). Prevertebral edema is also present (white arrow). B: Right parasagittal image (same sequence as A). The right C4 inferior facet is jumped and locked in front of the C5 superior facet (arrow). C: Unilateral facet dislocation at C3-4 in another patient. There is increased fluid within the joint capsule in the subluxed facet joint (white arrow). Note the incidental thrombosed right vertebral artery (dotted arrows). (Figure 8-21A,B from Flanders AE, Croul SE. Spinal trauma. In: Atlas SW. Magnetic Resonance Imaging of the Brain and Spine. 3rd ed. Philadelphia: Lippincott Williams & Wilkins; 2002:1781, with permission.) |
Unlike the ALL, the PLL is much more variable in width. The PLL is widest at the level of the intervertebral disc and thinner as it passes behind the vertebral bodies (54). Therefore, the PLL may normally appear discontinuous on sagittal MRI images (14). The PLL is represented on MRI as a thin, hypointense band that is interposed between the ventral dural sac and the posterior margin of the vertebral bodies and intervertebral discs. The PLL is the principal ligament of the middle column, which includes the posterior one half of the vertebral body and the annulus fibrosis. The PLL is best visualized on T2-weighted and intermediate-weighted sagittal images; however, the PLL is often impossible to resolve as a separate structure from the ventral dura or annulus on midsagittal images. The PLL is better delineated when elevated away from the posterior cortex by a herniated disc or posttraumatic fluid collection (Figs. 8-19, 8-20, 8-21 and 8-22, 8-24, 8-25 and 8-26, 8-29). As with the ALL, rupture of the PLL is identified as a focal region of discontinuity. Typically, rupture of the PLL occurs in hyperflexion and hyperextension type injuries.
The LF forms a continuous strip of fibroelastic tissue that bridges adjacent lamina. Along with the ISP, they act as check ligaments to oppose hyperflexion and distraction of the posterior elements and maintain alignment. They
are the principal ligaments of the posterior column, which includes all of the posterior elements. Normally, the LF are small structures (especially in the cervical and thoracic regions), which are oriented parallel to the adjacent lamina. Focal discontinuity of the LF can be identified on the parasagittal MRI (Figs. 8-30, 8-32). The LF may enlarge either on a degenerative basis or physiologically by bulging into the spinal canal in hyperextension. LF rupture is often associated with fractures of the posterior elements. The injured LF can be easier to visualize when the damaged segment projects into the spinal canal and distorts the posterolateral aspect of the thecal sac. This finding is best seen on parasagittal and axial images (Figs. 8-19, 8-20 and 8-21, 8-23, 8-24, 8-25 and 8-26, 8-29, 8-30, 8-31 and 8-32).
are the principal ligaments of the posterior column, which includes all of the posterior elements. Normally, the LF are small structures (especially in the cervical and thoracic regions), which are oriented parallel to the adjacent lamina. Focal discontinuity of the LF can be identified on the parasagittal MRI (Figs. 8-30, 8-32). The LF may enlarge either on a degenerative basis or physiologically by bulging into the spinal canal in hyperextension. LF rupture is often associated with fractures of the posterior elements. The injured LF can be easier to visualize when the damaged segment projects into the spinal canal and distorts the posterolateral aspect of the thecal sac. This finding is best seen on parasagittal and axial images (Figs. 8-19, 8-20 and 8-21, 8-23, 8-24, 8-25 and 8-26, 8-29, 8-30, 8-31 and 8-32).
Disruption of the ISP is best appreciated on the fat-suppressed midsagittal T2-weighted views. Fat suppression is essential for the detection of the typical high signal intensity in the tissues interposed between the widened spinous processes (Figs. 8-19, 8-21, 8-31, 8-33) (16). The supraspinous ligament forms a long contiguous band connecting the tips of the adjacent spinous processes and also serves as a posterior tension band that resists hyperflexion. The ruptured free edge of this structure may be visible within the edematous posterior paraspinal soft tissues (Fig. 8-19).
The facet joint complexes are easily identified on sagittal and axial images, particularly in the cervical and lumbar region where the structures are somewhat larger in size and the joint plane is oriented in the sagittal direction. In the thoracic spine, the facet joints are small in size and the joint is oriented in the coronal plane.
The facet joint complex is a dynamic structure that permits limited compression and distraction of the posterior elements during extension and flexion while resisting rotation and translation. Although fractures that involve the facet surfaces are better detected with tomography and CT, subtle damage to the synovial capsule and cartilaginous surface of the joint is best appreciated
with MRI. The facets are demonstrated on the far left and right parasagittal images of a well-centered sagittal sequence. The articular surface of the superior facet normally maintains close apposition to the inferior facet surface. Widening of this space is suggestive of a distraction injury (Fig. 8-24). The imaging criteria of altered facet alignment or subluxation are similar to the plain film appearance (Figs. 8-20, 8-21, 8-24, 8-29, 8-33, 8-34). Increased fluid within the joint space is suggested by a well-demarcated hyperintense focus interposed between the articular surfaces on T2-weighted and GE images (Fig. 8-21). The increased fluid is contained within the joint space and joint capsule.
with MRI. The facets are demonstrated on the far left and right parasagittal images of a well-centered sagittal sequence. The articular surface of the superior facet normally maintains close apposition to the inferior facet surface. Widening of this space is suggestive of a distraction injury (Fig. 8-24). The imaging criteria of altered facet alignment or subluxation are similar to the plain film appearance (Figs. 8-20, 8-21, 8-24, 8-29, 8-33, 8-34). Increased fluid within the joint space is suggested by a well-demarcated hyperintense focus interposed between the articular surfaces on T2-weighted and GE images (Fig. 8-21). The increased fluid is contained within the joint space and joint capsule.
Disc Injury
The identification and classification of a traumatic disc injury are important factors in determining the timing of and type of surgical decompression and stabilization (59, 60). Although posttraumatic disc herniation does not correlate with the degree of associated injuries or neurologic deficit, unrecognized disc herniation is a cause of neurologic deterioration after stabilization (8, 59, 61). Formerly, myelography, noncontrast CT, and CT myelography played an important role in determining whether extruded disc material compromised the epidural space and the thecal sac. Noncontrast CT is relatively insensitive to disc herniation as compared to MRI; however, the high resolution and isotropic data sets produced with MDCT often routinely depict disc herniations and the soft tissue contents of the spinal canal (8, 10, 49).
Although degenerative disc herniations are probably more common in the lumbar spine, posttraumatic disc herniations are encountered more frequently in the cervical and thoracic regions (17, 53, 59). In the cervical region, disc herniations most commonly occur at the C4-7 levels (8, 62). The incidence of posttraumatic thoracic
disc herniations are more common than previously estimated; they occur in up to 50% of thoracic injuries (59). When only single detector CT and CTM, were available, for the evaluation of spine trauma, cervical disc herniations were estimated to occur in only 3% to 9% of all cervical spine injuries. In addition, a large number of false-positive cervical disc herniations were reported using CT and myelography alone (59). With the routine use of MRI, the reported incidence of cervical disc herniation is reported as high as 54% (8, 60, 61 and 62). Cervical disc herniations were associated with 80% of bilateral facet dislocations, 60% of hyperextension injuries, 47% of central cord injuries, and all cases of anterior cord syndromes (60). Twenty-two percent of neurologically normal patients demonstrated disc herniations on MRI (62). Cervical disc herniation is reported to occur more frequently in flexion-distraction and flexion-compression-type injuries (63). The existence of thecal sac compression by herniated disc material is a significant factor in determining whether a discectomy should be performed at the time of surgical stabilization (60, 63). In addition, residual spinal cord compression from a posttraumatic disc herniation is associated with more severe neurologic injuries than disc herniation without cord compression (8, 64, 65). However, in a study by Dai and Jai (60), the authors found no significant relationship between neurologic deficit or neurologic recovery rate and severity of spinal cord compression by herniated disc material. Nevertheless, the authors concluded that surgical management is advised when residual spinal cord compression is demonstrated on MRI.
disc herniations are more common than previously estimated; they occur in up to 50% of thoracic injuries (59). When only single detector CT and CTM, were available, for the evaluation of spine trauma, cervical disc herniations were estimated to occur in only 3% to 9% of all cervical spine injuries. In addition, a large number of false-positive cervical disc herniations were reported using CT and myelography alone (59). With the routine use of MRI, the reported incidence of cervical disc herniation is reported as high as 54% (8, 60, 61 and 62). Cervical disc herniations were associated with 80% of bilateral facet dislocations, 60% of hyperextension injuries, 47% of central cord injuries, and all cases of anterior cord syndromes (60). Twenty-two percent of neurologically normal patients demonstrated disc herniations on MRI (62). Cervical disc herniation is reported to occur more frequently in flexion-distraction and flexion-compression-type injuries (63). The existence of thecal sac compression by herniated disc material is a significant factor in determining whether a discectomy should be performed at the time of surgical stabilization (60, 63). In addition, residual spinal cord compression from a posttraumatic disc herniation is associated with more severe neurologic injuries than disc herniation without cord compression (8, 64, 65). However, in a study by Dai and Jai (60), the authors found no significant relationship between neurologic deficit or neurologic recovery rate and severity of spinal cord compression by herniated disc material. Nevertheless, the authors concluded that surgical management is advised when residual spinal cord compression is demonstrated on MRI.
Posttraumatic disc changes on MRI can be classified as either disc injury or disc herniation. Normally, the well-hydrated intervertebral disc is hypointense relative to bone marrow on T1-weighted images and intermediate in signal on T2-weighted FSE images. The nondegenerated disc is uniform and symmetric in height, and
the peripheral fibers of the annulus fibrosus merge imperceptibly with the longitudinal ligaments. Disc injury is implied whenever there is asymmetric narrowing or widening of an isolated disc space on sagittal images and focal hyperintensity of the disc material on T2-weighted images. The injured disc is often higher in signal intensity than the adjacent discs on T2-weighted images, and the level of injury is usually contiguous with other damaged tissues (Figs. 8-21, 8-25, 8-28, 8-33). The observed signal changes in the disc may be the result of tearing of the disc substance during hyperflexion, hyperextension, or subluxation (8, 14, 53). As the adult intervertebral disc is an avascular structure, the observed, potentially hemorrhagic MR signal changes of a damaged disc may therefore be, in part, due to damage to the adjacent endplates. The signal changes of the injured disc may be easier to identify in patients with hypointense discs from pre-existing degenerative disc disease (Fig. 8-28).
the peripheral fibers of the annulus fibrosus merge imperceptibly with the longitudinal ligaments. Disc injury is implied whenever there is asymmetric narrowing or widening of an isolated disc space on sagittal images and focal hyperintensity of the disc material on T2-weighted images. The injured disc is often higher in signal intensity than the adjacent discs on T2-weighted images, and the level of injury is usually contiguous with other damaged tissues (Figs. 8-21, 8-25, 8-28, 8-33). The observed signal changes in the disc may be the result of tearing of the disc substance during hyperflexion, hyperextension, or subluxation (8, 14, 53). As the adult intervertebral disc is an avascular structure, the observed, potentially hemorrhagic MR signal changes of a damaged disc may therefore be, in part, due to damage to the adjacent endplates. The signal changes of the injured disc may be easier to identify in patients with hypointense discs from pre-existing degenerative disc disease (Fig. 8-28).
An acute, posttraumatic disc herniation has a similar MRI appearance to nontraumatic disc herniation. The nucleus pulposus is forced under pressure to extrude into the peripheral annulus fibrosus and, in some instances, extend beyond the outer annulus into the anterior epidural space. The herniation may be broad-based or eccentric and may or may not be associated with a vertebral body fracture. On a sagittal MRI, the disc herniation is isointense and contiguous with the disc of origin (2, 8, 10, 12, 14, 18). A small herniated disc fragment often appears as a focal area of expansion of the annulus beyond the border of the posterior cortical margin (Figs. 8-21, 8-29, 8-35, 8-36). Occasionally, a small rent in the annulus may appear that allows passage of nuclear material into the epidural space. On axial images, the herniated disc produces focal distortion of the ventral theca (Fig. 8-35). Depending on the size and location of the disc herniation, the fragment may be demonstrated on multiple sagittal and axial images.
The degree of compressive injury to the neural elements depends on the size of the herniated fragment, the width of the spinal canal at the level of injury, and the
diameter of the spinal cord. For example, a small disc herniation in the thoracic region may cause more neural impingement than an identical fragment would cause in the lumbar or cervical regions (66).
diameter of the spinal cord. For example, a small disc herniation in the thoracic region may cause more neural impingement than an identical fragment would cause in the lumbar or cervical regions (66).
![]() FIGURE 8-28. Extension mechanism injury at C5-6. A: Sagittal FSE T2-weighted image shows changes of multi-level degenerative cervical spondylosis and stenosis spanning C3-6. There is acute widening of the anterior aspect of the C5-6 disc space (curved arrow) and the disc material is hyperintense secondary to injury. Spinal cord edema is also present. B: Axial GE axial image obtained through the C5-6 interspace shows the retropulsed spondylotic disc fragment (arrow) compressing the thecal sac. C: Sagittal T2 weighted image in a different patient shows extension type injury at two distinct levels (C4-5 and C6-7). Note the anterior widening of the interspaces with increased signal in the damaged discs (arrows). There is a large prevertebral hematoma (asterisk). (Figure 8-28A,B from Flanders AE, Croul SE. Spinal trauma. In: Atlas SW. Magnetic Resonance Imaging of the Brain and Spine. 3rd ed. Philadelphia: Lippincott Williams & Wilkins; 2002:1784, with permission.) |
Identification of an acute disc herniation can be difficult in the setting of superimposed degenerative spondylotic changes (17). In such instances, multiple chronic spondylotic disc herniations associated with osteophytes may complicate the correct identification of an acute traumatic disc herniation (24). Imaging factors that may aid in the identification of an acute disc herniation with superimposed spondylosis include alteration in signal of the disc material at one level, asymmetric width of the intervertebral disc space, subluxation, and associated injuries at the same level (Fig. 8-28). In some circumstances, definitive identification may be impossible.
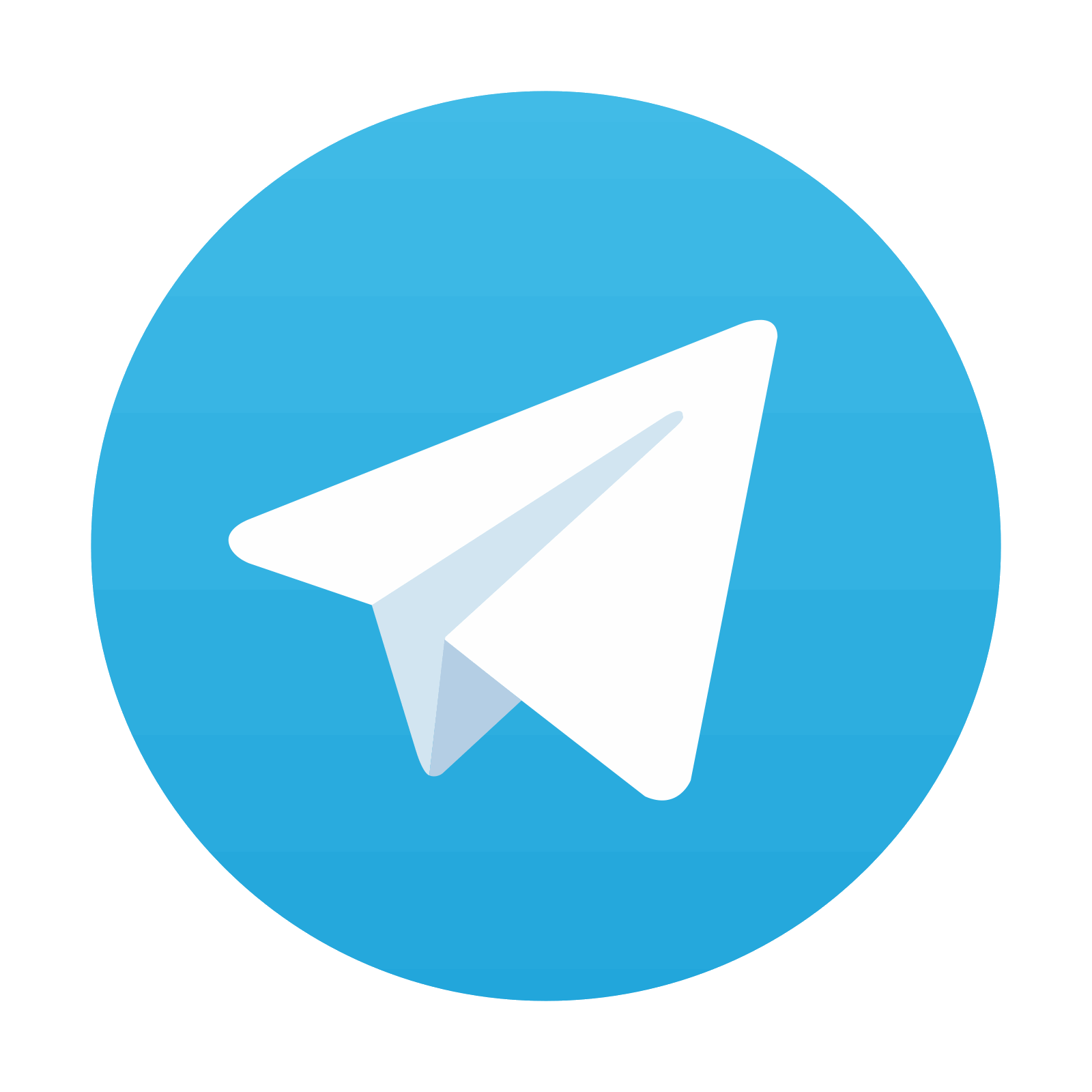
Stay updated, free articles. Join our Telegram channel
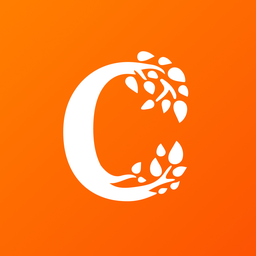
Full access? Get Clinical Tree
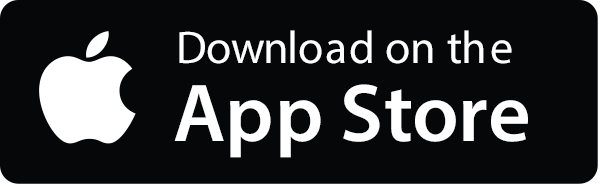
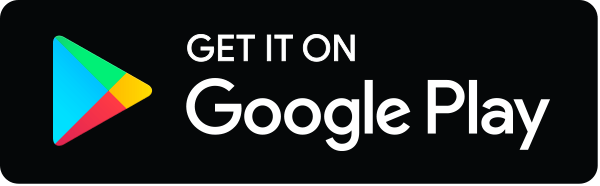