and Robert E. Schmidt2
(1)
Sunnybrook and St Michael’s Hospitals, University of Toronto, Toronto, ON, Canada
(2)
Division of Neuropathology Department of Pathology, Washington University School of Medicine, St. Louis, MO, USA
In this chapter, we review the peripheral nerve anatomy pertinent to interpretation of biopsy material. We discuss the epineurial, perineurial, and endoneurial compartments of nerve, followed by a description of the changes that occur with aging. In subsequent chapters, we will deal with the ultrastructure and pathophysiology of axons, Schwann cells and myelin, the neural vasculature, as well as aspects of quantitative morphometry. Because the sural nerve is almost universally the nerve chosen for biopsy, certain elements of the discussion are specific to this site.
2.1 Normal Peripheral Nerve Structure and Function
2.1.1 Macroscopic Anatomy of the Sural Nerve
The majority of axons that form the sural nerve have their cell bodies in the S1 spinal dorsal root ganglia. However, electrophysiological techniques indicate that L5 and S2 roots also make significant contributions, and, in rare cases, even L3 and L4 contribute as well (Phillips and Park 1993; Liguori et al. 1992). Axons exit through the dorsal roots and travel in the lumbosacral trunk to the sciatic nerve. Most of these fibers travel in the medial division of the sciatic nerve, which goes on to form the tibial nerve (Fig. 2.1). A lesser number travel in the lateral division of the sciatic nerve, which becomes the peroneal nerve. In the popliteal fossa, fibers branch from the tibial nerve to form the medial sural cutaneous nerve. At the same level or slightly below, the peroneal nerve gives rise to the lateral sural cutaneous branch, most of which does not contribute to the sural nerve proper. Rather, the peroneal communicating branch of the lateral sural cutaneous nerve usually joins with the medial cutaneous sural nerve to form the sural nerve proper (Fig. 2.1). The point of union between these two components defines the beginning of the sural nerve. In 80 % of patients, this union occurs 11–20 cm proximal to the lateral malleolus. In 20 %, the sural nerve is entirely derived from the posterior tibial nerve (Ortiguela et al. 1987). Rarely, the sural nerve may arise entirely from the common peroneal nerve (Phillips and Park 1993). In up to 5 % of cases, no junction develops between the medial cutaneous sural nerve and the peroneal communicating nerve, resulting in two separate trunks, both smaller than normal (Behse et al. 1974). The sural nerve subsequently courses laterally and distally, passing 1–1.5 cm posterior to the lateral malleolus adjacent to the lesser saphenous vein. Beginning 2–3 cm distal to the lateral malleolus, cutaneous branches diverge from the main trunk. Two or three cutaneous branches may arise proximal to the lateral malleolus (Ortiguela et al. 1987).


Fig. 2.1
Normal anatomy of the sural nerve, right leg
Approximately 20 % of people possess an accessory deep peroneal nerve that supplies motor innervation to the extensor digitorum brevis (Lambert 1969; Infante and Kennedy 1970). When present, this nerve ordinarily runs just anterior to the sural nerve behind the lateral malleolus. Typically, the sural and the accessory deep peroneal nerve fascicles are separate nerve trunks. Singular reports of these motor fibers running in the sural nerve are unconfirmed by larger series (Lambert 1969). On occasion, we have received nerve biopsy specimens containing only a few fascicles and showing an unusual distribution of myelinated fibers. We suspect that in these situations an accessory peroneal nerve was biopsied or, alternatively, that a non-joined medial cutaneous sural nerve or peroneal communicating branch was taken instead of a whole sural nerve (Behse et al. 1974).
The sural is a pure sensory nerve, supplying the skin over the lateral and posterior ankle and lateral foot to the base of the fifth toe, as well as various components of the ankle joint. Although the presence of autonomic fibers is often assumed (e.g., as cutaneous sudomotor or vasoconstrictor fibers), authors who have pursued the issue have not found any evidence to suggest that these fibers make significant contributions to the sural nerve at this location (Chad et al. 1981; Sjo et al. 1976).
2.1.2 The Microscopic Architecture of Nerves
2.1.2.1 Epineurium
The epineurium constitutes approximately half of the cross-sectional area of a nerve trunk and consists predominantly of connective tissue that holds together and protects the individual fascicles forming the nerve. Normally, the sural nerve (Fig. 2.2) comprises between 6 and 15 fascicles, but investigators have occasionally observed more than 20. Collagen bundles run predominantly longitudinally along the nerve and are packed more closely immediately adjacent to the perineurium of individual nerve fascicles. Fibrous tissue is also packed more densely at the periphery of the nerve trunk, forming a sheath. Type I collagen predominates over type III in the epineurium (Shellswell et al. 1979), and the collagen fibrils formed are 60–110 nm in diameter. Adipocytes intermingle with the fascicles in variable amounts. Elastic fibers have a longitudinal orientation.


Fig. 2.2
The normal sural nerve: Semithin cross section of entire specimen, composed of ten fascicles surrounded by a thin perineurium which blends imperceptibly into the surrounding epineurium. The large green epineurial bodies are adipocytes. (1 μ thick toluidine blue-stained plastic section)
Although the epineurium contains a lymphatic drainage system, from a pathologist’s perspective the most important structures found in this site are capillaries, arterioles, and venules. In Chap. 6, we discuss neural vascular anatomy in greater detail. It is noteworthy that occasionally a thin cuff of lymphocytes can be seen around small epineurial vessels in normal nerve and noninflammatory neuropathies of uncertain significance. Other cell types normally seen in the epineurium include fibroblasts and mast cells. Not appreciated on routine microscopy is a neural meshwork (the nervi nervorum) found within the epineurium and perineurium, presumably involved in vasomotor control and some sensory perception (nerve infarction is locally painful) (Beggs et al. 1991). An occasional event is the finding of epineurial (Fig. 2.3a) or endoneurial (Fig. 2.3b) Pacinian corpuscles in the sural nerve. The literature also contains reports of other unusual sensory corpuscles within the endoneurial compartment (Hall et al. 1991).


Fig. 2.3
Pacinian corpuscles in the epineurium (a) and endoneurium (b) are rare findings of no pathological significance. (1 μ thick toluidine blue-stained plastic sections)
2.1.2.2 Perineurium
On cross sections, the perineurium (reviewed in detail in Piña-Oviedo and Ortiz-Hidalgo 2008) appears as a lamellated structure that surrounds all nerve fascicles (Figs. 2.2 and 2.4a). If the circular lamellae are “unrolled,” perineurial cells will be seen as flattened polygonal cells, attached to each other as in patches of a quilt (Olsson 1990). The number of perineurial lamellae varies directly with the size of the fascicle (Tohgi et al. 1977). Distal nerve fascicles in intramuscular nerves have only one perineurial cell layer, large nerve trunks may have up to 15 layers; typical sural nerve fascicles usually show 5–8 lamellae. The outer perineurial layers merge with the fibroblasts and collagen of the epineurium.


Fig. 2.4
Perineurium: in (a) the blending of perineurial layers without intervening epineurium occurs as the two fascicles merge. In (b) delicate perineurial calcifications (arrow) are shown. (1 μ thick toluidine blue-stained plastic sections)
Perineurial cells are invariably surrounded by a basement membrane, typically thicker than that seen around Schwann cells or vessels (Fig. 2.5a–c). Each perineurial layer is one cell wide except at sites where adjacent cells overlap or interdigitate (Fig. 2.5c). A cleft containing collagen separates these layers. In cross section, perineurial cells sometimes appear to branch and join an adjacent layer. The cytoplasm of these cells is rarefied except in the perinuclear region (Fig. 2.5c) where organelles such as mitochondria, glycogen granules, and endoplasmic reticulum occur. The nucleus is elongated and sometimes multilobulated. Perineurial cells also contain filaments reminiscent of those seen in smooth muscle fibers. A prominent feature is a large number of smooth contoured pinocytotic vesicles in various stages of fusion with the cell membrane (Fig. 2.5a). Transmission electron microscopy demonstrates that tight junctions (zonula occludens, arrows, Fig. 2.5a, c) link adjacent perineurial cells, and freeze fracture studies show that these junctions are arranged in numerous long branched arrays (Beamish et al. 1991). This suggests that the perineurium has a barrier property, a hypothesis supported by functional studies of the blood–nerve barrier. Gap junctions may occur between adjacent perineurial cells only rarely (Beamish et al. 1991).


Fig. 2.5
Typical perineurial cells showing tight junctions (arrows) and pinocytotic vesicles. Longitudinal and circumferential networks of collagen are seen between the perineurial cell layers. Note clumps of oxytalan (arrowhead) (a, b: 26,400×; c: 40,000×)
Between the leaves of the perineurium lies a meshwork of collagen fibrils with longitudinal, circumferential, and oblique orientations. At 40–64 nm, the diameter of these fibrils is visibly less than that of epineurial collagen (Fig. 2.5b). The smaller size of these collagen fibrils and immunohistochemical studies suggest that type III collagen is an important constituent, although type I collagen is also present (Lorimier et al. 1992; Shellswell et al. 1979). Tangles of non-branching filaments 10–12 nm in diameter are also seen, the latter probably representing what has been termed oxytalan, a fibrillar precursor of elastic fibers (vide infra). The occasional fibroblast may be detected. Long-spaced collagen (“Luse bodies”) (Fig. 2.6a) and occasionally perineurial calcifications (Fig. 2.6b) can be seen in normal individuals. Such calcifications increase with age and can also be a dramatic but a nonspecific feature of peripheral neuropathies (Figs. 2.4b and 2.6b).


Fig. 2.6
“Raccoon tail” (long-spaced collagen, Luse body) is seen between perineurial cells (a). Osmiophilic bodies, some concentrically layered, represent perineurial calcifications (b). (a: 30,420×; b: 12,070×)
Cross-sectional examination of nerve fascicles often reveals partitions or septae which subdivide the endoneurium. These partitions are made up of perineurial cells, and if followed distally or proximally, these subdivisions of endoneurial contents will be seen to demarcate the sites where fascicles join or branch off (Figs. 2.4a and 6.4).
Perineurial cells are derived from fibroblasts (Bunge et al. 1989), and the perineurial layers which surround nerve fascicles are continuous with the fibroblasts in the pia mater surrounding the nerve roots (Waggener and Beggs 1967; Piña-Oviedo and Ortiz-Hidalgo 2008). It is thought that a chemical mediator, likely desert hedgehog derived from Schwann cells, induces and maintains differentiation of fibroblasts into perineurial cells and directs their organization (Thomas and Bhagat 1978; Piña-Oviedo and Ortiz-Hidalgo 2008) as perineurial cells influence Schwann cell basement membrane structure. Neurofibromin may also play a role in perineurium formation. Perineurial cells have been observed to assume characteristics of fibroblasts when intrafascicular contents are extracted and return to their previous morphology when neural structures regenerate (Thomas and Bhagat 1978). When the perineurium is stripped from living nerve, its regeneration occurs through the migration of endoneurial fibroblasts to the periphery, and their subsequent differentiation into typical perineurial cells (Nesbitt and Acland 1980).
The perineurium is thought to play an important role in regulating the endoneurial milieu in ways similar to the blood–nerve barrier. Perineurial cells have the necessary complement of enzymes to permit a high degree of metabolic activity (Olsson 1990). Experiments with a variety of dyes and protein tracers have indicated that most macromolecules cannot pass the innermost layer of the perineurium (Olsson 1990), while electrolytes and glucose can pass through slowly. Although investigators have not clearly established the role of the numerous pinocytotic vesicles seen in perineurial cells, the vesicles may act in maintaining the special osmolar and molecular composition of endoneurial fluid. Interestingly, pinocytotic vesicles are more abundant in the external perineurial sheaths with more prominent cell junctions in the inner perineurial layers (Reina et al. 2003). In rapidly evolving neuropathies, debris-filled macrophages often accumulate in the subperineurial area, and debris may appear within perineurial cells in both experimental models (de la Motte et al. 1975), and in human peripheral nerve disease.
2.1.2.3 Endoneurium
The endoneurium consists of the region internal to the innermost layer of the perineurium, excluding perineurial partitions within the fascicle and including all cellular and extracellular components. We will review the fine structure and function of axons, Schwann cells, and the neural vasculature in subsequent chapters.
The endoneurial compartment is under pressure relative to the epineurium, as demonstrated by the tendency of endoneurial contents to herniate out of a perineurial window (Spencer et al. 1975). Technically difficult to accomplish, measurements of endoneurial pressure range from 0.4 to 2.7 mmHg (Low et al. 1977). This expansile tendency and the elastic properties of perineurium create the uniformly circular shape of each fascicle (Sunderland 1978), and any deviation from circularity, except at branching points, indicates either an artifact or a sign of pathology.
The distal sural nerve has a total endoneurial cross-sectional area of 0.65–1.26 mm2 when Renaut bodies are excluded (Behse 1990), with individual fascicles ranging in size from 0.02 to 0.46 mm2 (Prineas and McLeod 1976). Myelinated fibers and their Schwann cells account for 24–36 % of this total cross-sectional area, and unmyelinated fibers and their investing Schwann cells for 11–12 % (Behse 1990). The other major component of cross-sectional area is the interstitial compartment which includes densely packed collagen (35–45 %, ranging from 30 to 65 nm) and optically empty interstitial space presumably containing water and macromolecules (13–14 %). Vessels account for 2–3 % of the endoneurial area in the rat (Bell and Weddell 1984), although this area may be less than 1 % in man (Behse et al. 1974).
Semithin light microscopic sections (Fig. 2.7a, b) and low-power electron microscopy (Fig. 2.8) best reveal the organization of axons and Schwann cells and their interrelationship in the endoneurium. The superior light microscopic resolution of plastic embedded toluidine blue-stained semithin (1 μm) sections (Fig. 2.7a, b) allows rapid identification of axons and myelin. Even the best paraffin sections cannot rival this technique. Two populations of axons are identified, myelinated fibers (MFs) or unmyelinated fibers (UFs). The latter are best studied with electron microscopy.



Fig. 2.7
The sural nerve of a young man. Fiber density and myelination are normal. The axons contain punctate densities, which represent mitochondria. Unmyelinated fibers appear in clusters, often associated with MFs of small diameter, and segregated from large MFs. In (b), except for occasional fibroblasts (arrows), most nuclei belong to Schwann cells. (1 μ thick toluidine blue-stained plastic sections, a: 200×, b: 1,000×)

Fig. 2.8
The normal sural nerve of a 25-year-old woman. Denervated Schwann cells (arrows) and collagen pockets (arrowhead) are normally present (3,520×)
2.1.2.4 Myelinated Axons
Apart from a small area just beneath the perineurium that remains cell-free, semithin sections show an even dispersion of myelinated fibers of various calibers throughout the endoneurium. Casual inspection indicates that MFs have a bimodal size spectrum. Tissue sections from human material rarely show the perfectly round outlines of MFs present in specimens from experimental animals after perfusion fixation. Myelin and axons are fragile structures with a liquid-gelatinous consistency. Consequently, the stresses inflicted upon the sural nerve as it is exposed, cut, and fixed by immersion, result in a loss of this circularity. With toluidine blue staining, the axon appears as a sharply demarcated lightly stained area, surrounded by its homogenously dark myelin sheath. A few punctate regions of staining within the axon most often represent mitochondria. Each myelinated axon segment is associated with only a single Schwann cell. This axonal segment and its associated myelin sheath define the “internode,” which varies between 200 and 1,800 μm in length, depending mostly on the axon’s diameter and whether pathology is present. Most cross sections through an internode show compact myelin surrounding the axon; however, a cut through the center of an internode will reveal the nucleus of the Schwann cell that is associated with the axon and maintains its myelin. In one study, 9 % of small myelinated and 3 % of large myelinated internodes were cut through the nuclear region, reflecting the relatively constant length of Schwann cell nuclei, and the fact that internodes of large myelinated fibers are about three times longer than those of small myelinated fibers (Ochoa and Mair 1969; Behse 1990).
The node of Ranvier, which is the site where adjacent internodes meet, is a 1 μm long region where no myelin surrounds the axon. The node and its molecular constituents have been extensively studied and reviewed (see Scherer and Wrabetz 2008), which is particularly relevant to inherited myelinopathies and CMT. On cross sections, an axon is rarely cut precisely at the node, but when this cut does occur, it should not be mistaken for a demyelinated axon. The increased staining density of axoplasm at the node of Ranvier and paranodal axon is the most reliable clue to this occurrence (Fig. 4.1c, d). The axoplasm lacks the cellular structures associated with protein synthesis, i.e., large numbers of ribosomes (although individual ribosomes have been reported in normal and pathological axons), rough endoplasmic reticulum, and Golgi apparatus. These features, in conjunction with the length of peripheral nerve axons, have significant consequences for their growth, long-term maintenance, and the mechanisms of axoplasmic transport.
The predominant component of the axoplasm is an electron-lucent matrix within which is suspended a population of filaments and organelles. The smallest constituents consist of microfilaments measuring 5–7 nm in diameter. They contribute approximately 10 % to the total complement of axonal protein. Their numbers are underestimated in conventional transmission electron microscopy studies because the stability of microfilaments is severely compromised by the destructive effects of glutaraldehyde and osmium tetroxide. Neurofilaments, far more obvious common components of axoplasm, consist of longitudinally orientated filaments of 8–11 nm in diameter and indefinite length. The neurofilament density remains constant along the length of individual fibers and is not affected by axonal diameter. Neurofilaments are well preserved after the use of aldehyde fixatives, but they are susceptible to digestion by an intrinsic axonal calcium-activated protease which may account for the effacement of neurofilaments from damaged axons. Clusters of neurofilaments may account for the visibility of axoplasmic and cytoplasmic fibrillar structures which develop after metallic impregnation (e.g., Bielschowsky silver stain). The term microtubules (“neurotubules”) defines larger diameter cytoskeletal structures in the axoplasm. Singly or in parallel arrays, they are unbranched longitudinally oriented, hollow structures with a diameter of 23–25 nm. Microtubules consist of globular subunits of the protein tubulin arranged in a lattice and are labile structures with a proclivity to disaggregate when exposed to colchicine and related mitotic spindle inhibitors. The sum of the numbers of the microtubules in the terminal branches of large axons is considerably larger than the number found proximally. A relatively larger density of microtubules is found in unmyelinated axons in comparison to myelinated axons. The axoplasm also contains various types of membranous organelles comprising mitochondria, smooth endoplasmic reticulum, lysosomes, vesicles, and dense-core vesicles of a range in sizes. Coated vesicles occur at the axolemma of myelinated and unmyelinated fibers, particularly at the neuromuscular junction and at the nodes of Ranvier.
Investigators have studied the fiber composition and quantitative morphometry of the sural nerve more intensely than any other nerve and thus quantitative analysis of biopsies from this site is most reliable. However, most of the information pertaining to the general organization of peripheral sensory nerves is widely applicable. The quantitative data provided below is derived from a single laboratory (Behse 1990; Behse et al. 1974); however, these numbers are representative of the literature. Chapter 3 provides a broader discussion of issues surrounding quantitation of nerve biopsy findings.
Myelinated fibers show a bimodal size distribution. When defining axonal size, the investigator needs to specify either the axonal diameter or diameter of the axon plus its myelin sheath. Most workers use the latter approach, and unless otherwise specified, this book will adhere to this convention. Fiber diameters form a spectrum with some overlap between large and small MFs. Typically, small MFs range from 2 to 6 μm in diameter (axon plus myelin), and large MFs range from 8 to 12 μm in diameter, with peaks at 4 and 10 μm, respectively. Using 7 μm as the separation point between large and small MFs, about 32–45 % are large and 55–68 % are small. If one considers only the axonal (i.e., inner myelin) diameters of MFs (for purposes of comparison with unmyelinated fibers), small myelinated axons range in size from 1 to 3 μm, and large axons from 3 to 6 μm, rarely as large as 7–8 μm.
The absolute number of MFs in the sural nerve at the lateral malleolus ranges from 5,200 to 9,460, while the MF density is 5,200–8,000/m2 (Behse 1990). It is difficult to know whether absolute fiber count or fiber density is the optimal measure. The former is probably subject to greater normal interindividual anatomical variability, while the latter is difficult to interpret in cases where fascicular size alterations are part of the pathology and can vary with fixation technique. In practical terms, however, investigators find it easier to derive a density from counting fibers in several representative endoneurial areas.
The thickness of the myelin sheath is proportional to axon diameter and likely reflects a complex interplay of trophic substances and molecular events (Quintes et al. 2010; Pereira et al. 2012). The parameters governing this relation are somewhat different for large vs. small myelinated fibers. As a rough guide, however, the ratio of axon (without its myelin) diameter to the fully myelinated axon diameter is normally 0.5–0.7. This has been called the “G-ratio” and is best assessed on ultrathin EM sections. Toluidine blue-stained light microscopic sections have a tendency to magnify the myelin sheath thickness relative to the axonal diameter.
Teased fiber preparations allow the measurement of internodal length (IL). As axon diameter increases, so does IL. For large myelinated axons, IL falls in the range of 0.35–1.83 mm, and for small myelinated axons in the range of 0.18–0.66 mm. Clearly, there is substantial variability within normal nerve, and this increases further with pathological processes and with aging.
2.1.2.5 Unmyelinated Axons and Their Schwann Cells
Unmyelinated fibers (UFs) are spread throughout the nerve fascicle, but have a preferential localization adjacent to small MFs, a relationship probably due to their shared developmental history (Figs. 2.7 and 2.8) (Thomas et al. 1993). Using oil-immersion light microscopy, unmyelinated axons appear as round clear areas within nonmyelinating Schwann cells. A myelin-producing Schwann cell never invests any axon other than the one around which its myelin is wrapped. On the other hand, Schwann cells associated with unmyelinated axons (historically called Remak cells and with their collections of axons, Remak bundles) may have several such fibers under their influence, with the Schwann cell cytoplasm wrapping around and entirely isolating each axon from its neighbors. Sharma and Thomas (1975) defined Schwann cell subunits (ScSu) as any single Schwann cell profile or group of profiles that in transverse section is enclosed by a continuous basal lamina (Sharma and Thomas 1975). This structure can include no axons to several axons. The usual number of clustered cross-sectional profiles per ScSu is 2–5, but as many as 20 profiles may be seen (Behse et al. 1975), and there are usually 1–4 UFs per ScSu. A ScSu lacking axons is referred to as “denervated.” ScSu lacking axons or containing collagen pockets are seen in normal nerves (Figs. 2.8 and 2.9) and increase with age and in neuropathy.


Fig. 2.9
Collagen pockets (arrows) surrounded by Schwann cell processes (21,840×)
Identifying the axon within a ScSu can prove difficult. Sometimes a Schwann cell will seem to partially or fully encircle a bundle of collagen fibers, forming a so-called collagen pocket (Fig. 2.9), which may mimic axons even with oil-immersion light microscopy of 1 μm thick toluidine blue-stained sections, although lacking punctate organelles visible in axons. Ultrastructurally, axons are recognized by their lesser electron density, greater microtubule density, and absence of ribosomes. The axolemma is also somewhat thicker than the Schwann cell membrane. Finally, a distinct mesaxon around a circular profile helps identify that profile as an axon. However, axons are not always completely and individually wrapped by Schwann cell processes, or have an identifiable mesaxon. Isolated projections of Schwann cell cytoplasm surrounded by basement membrane and without an associated axon occur in normal nerves as denervated ScSus or collagen pockets, increasing with age and disease (Behse et al. 1975; Behse 1990).
Unmyelinated fibers outnumber myelinated fibers by about 4:1. The normal variability in UF numbers is even greater than for myelinated axons, with a range of 20,100–51,350 per nerve and a density of 18,000–42,000/mm2. Unmyelinated axons have a unimodal size distribution, with fibers ranging in size from 0.1 to 3 μm, with a peak at 1–1.5 μm. There is, therefore, a degree of overlap of unmyelinated axon diameters with the smallest myelinated fibers. The only general features that distinguish UFs from MFs are their much higher proportion of axonal microtubules compared with neurofilaments, the frequent presence of dense-cored vesicles in autonomic efferent fibers, and a higher density of sodium channels. Rigorous quantitation and interpretation of unmyelinated fiber counts is complex. Although UFs are not segmented in the fashion of MFs, nonmyelinating Schwann cells do have a typical longitudinal extent of 200–500 μm (Carlsen et al. 1974).
2.1.3 Other Endoneurial Cell Content
The nucleated cells of the endoneurium are Schwann cells, fibroblasts, vascular endothelial cells, pericytes, smooth muscle cells, macrophages, lymphocytes, and mast cells. Quantitative assays of the numbers of these cells in the human sural nerve indicate that within the endoneurium about 80–90 % are Schwann cells and 10 % are fibroblasts, with nuclei of nonmyelinating Schwann cells outnumbering those of myelinating Schwann cells about 4:1 (Ochoa and Mair 1969). Endothelial cells are excluded from this count, and the other cell types are not present in significant amounts within the endoneurium, excepting perhaps macrophages (vide infra). There is interspecies and internerve variability in such statistics, probably relating to the differences in the unmyelinated fiber composition of the nerves examined (Thomas 1963).
2.1.3.1 Fibroblasts
Fibroblasts are the second most common nucleated endoneurial cell, accounting for about 10 % of cells, with a density of about 300 nuclei/mm2 (Ochoa and Mair 1969). These cells are thought to originate from neural crest stem cells and have a potential role in many endoneurial processes including reaction to injury including interleukin secretion, immune surveillance, and a supportive mechanical role (see Richard et al. 2012 for a detailed review). They are more prominent in the vicinity of endoneurial vessels. Use of light microscopy cannot always reliably distinguish fibroblasts from Schwann cells or native endoneurial histiocytes (Ochoa and Mair 1969). With immunohistochemistry, fibroblasts are variably immunoreactive for CD34 (Richard et al. 2012) and fail to stain with S-100 and EMA which distinguishes them from Schwann cells and perineurial cells, respectively. Fibroblasts may possess extremely thin and elongated cell processes (Fig. 2.10a, b). Ultrastructurally, they contain abundant granular endoplasmic reticulum, an irregular nucleus with a dense peripheral chromatin ring, and, most importantly, the absence of a surrounding basement membrane. Focal electron dense zones are sometimes present on the cell membrane and are highly characteristic. Small pinocytotic vesicles can often be seen just beneath the cell membrane. Frequently, fibroblasts occur in intimate contact with the collagen and oxytalan found ubiquitously in the endoneurium. A peculiar feature of some fibroblasts is “giant vacuolation” (Fig. 2.9a, b), especially within Renaut bodies (vide infra) and in regions of “edema”; however, it is uncertain whether these truly represent intracytoplasmic vacuolation or instead extremely convoluted cells caught in cross section.


Fig. 2.10
A vacuolated fibroblast is shown in the endoneurium in a one micron thick toluidine blue section (arrow, a) and by electron microscopy (b). (a: 1 μ thick toluidine-blue-stained plastic section, 1,000×; b: 6,000×)
2.1.3.2 Macrophages
Endogenous macrophages make up 2–9 % of the resident cell population of the endoneurium (Griffin et al. 1993). Their role in the peripheral nervous system has been reviewed (Griffin et al. 1993; Bonetti et al. 1993; Müller et al. 2006). Immunohistochemical techniques identify these cells reliably (Bonetti et al. 1993; Griffin et al. 1990) using antibodies against CD-68, Iba-1, and, even more specifically, CD-163. Quiescent (not activated) endoneurial macrophages are elongated cells which may demonstrate ramified spiny processes with their processes oriented along the longitudinal axis of the nerve. They do not possess a surrounding basement membrane and are often found in a perivascular distribution, stretched out in the longitudinal axis of the nerve (Griffin et al. 1993; Bonetti et al. 1993). Cross sections thus tend to de-emphasize their numbers, estimated at 50/mm2 in the human sural nerve (Bonetti et al. 1993). They are distinguished from fibroblasts by the presence of an inconspicuous rough endoplasmic reticulum, which is poorly populated with ribosomes, and by coated vesicles and dense granules. Pinocytotic activity is not a feature, although endocytosis into coated vesicles may occur. Residual macrophages are reported to undergo a physiological turnover of 50 % in the sciatic nerve within 12 weeks (Müller et al. 2010).
Investigation of this cell population has suggested several functions. Their location, ability to take up soluble macromolecules, dendritic organization of some of their cell processes, and their constitutive expression of MHC class II and CD4 antigens suggest a possible role as antigen-presenting cells (Griffin et al. 1993). Classic thinking postulated that tissue macrophages were incapable of significant reactive proliferation (Griffin et al. 1993), but endoneurial macrophages do proliferate and participate in experimental Wallerian degeneration (Hann-Bonnekoh et al. 1989; Müller et al. 2006). Consideration of different roles for endoneurial and circulating macrophages is complicated by the lack of unequivocal markers for each; however, studies with chimeric rodents and GFP-labeled cells have established roles for resident macrophages as early responders in Wallerian degeneration, genetic neuropathies, and inflammatory neuropathy (Müller et al. 2006). Further work by this laboratory suggests that resident endoneurial macrophages intrinsically generate the macrophage response in slowly progressive neuropathy, which is supplemented by hematogenous macrophages in distal areas of more pronounced damage (Müller et al. 2008). Nerve regeneration, inflammatory demyelination, and clearance and recycling of myelin lipids are some of the important processes in which the critical role of macrophages is increasingly appreciated (Griffin et al. 1993).
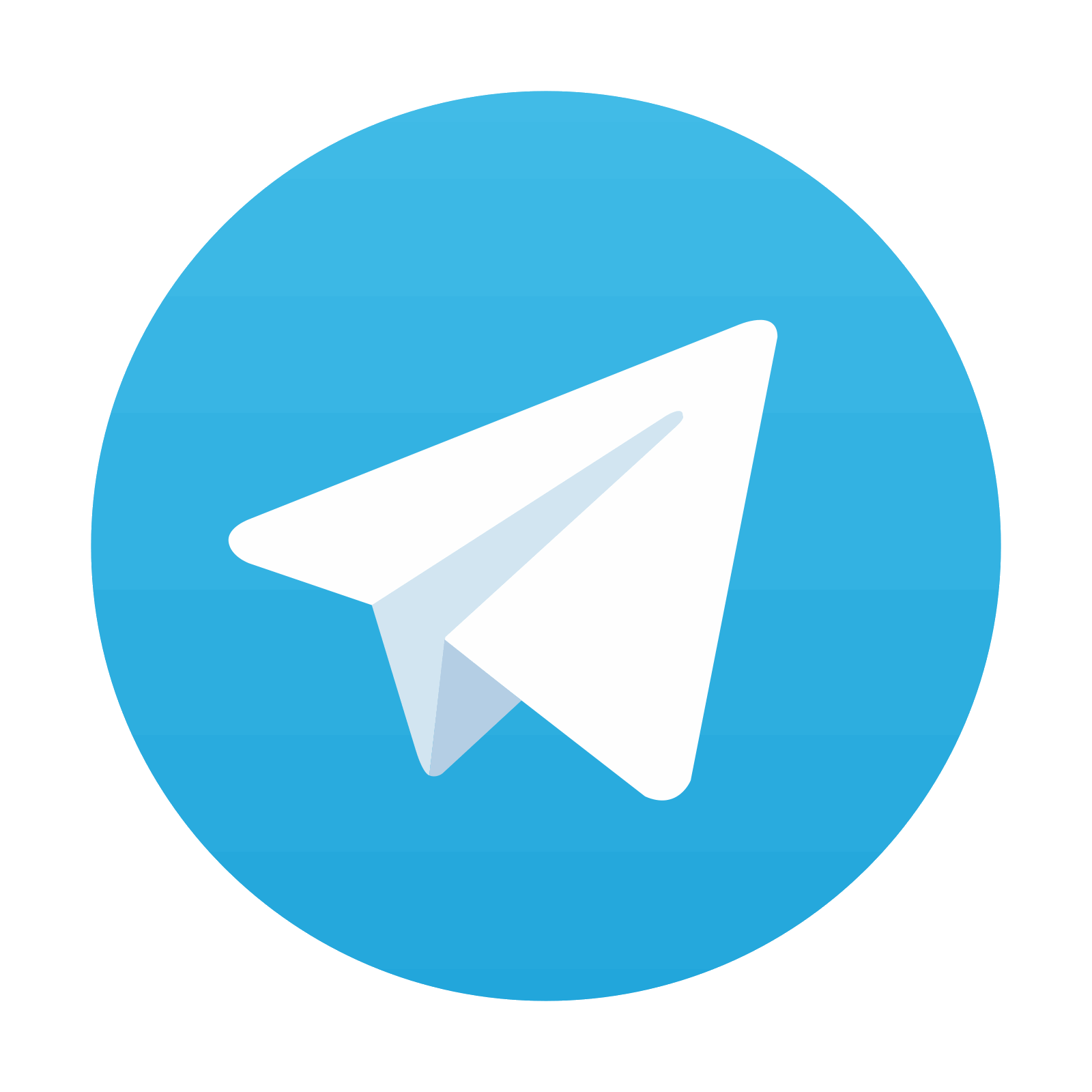
Stay updated, free articles. Join our Telegram channel
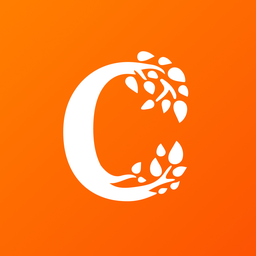
Full access? Get Clinical Tree
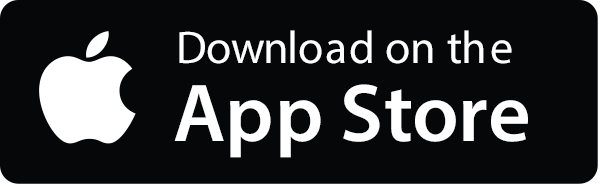
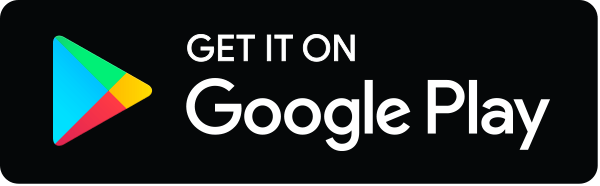
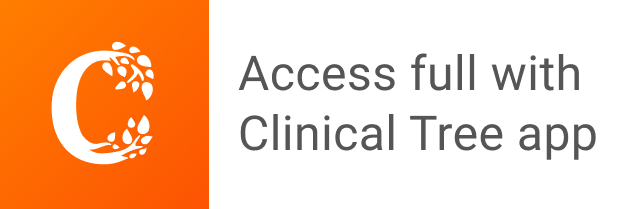