Abstract
The use of electricity for therapeutic purposes was first used almost 2000 years ago. Today, based on advancements in both electrophysiology and electromagnetic theory, numerous electromagnetic-based techniques and technologies have been developed to generate currents within the human nervous system to influence brain activity and behavior. In addition to electromagnetic techniques, acoustic, thermal, optical, and combined modalities have been proposed, all with various levels of success. In fact, despite their widespread use, even the most common noninvasive brain stimulation (NIBS) techniques, including transcranial magnetic stimulation (TMS) and transcranial direct current stimulation (tDCS), suffer from several limitations such as limited focality and penetration, which in turn limit their therapeutic effects. We will review the various NIBS modalities and present electrosonic stimulation, a novel form of NIBS with enhanced penetration, focality, and targeting control compared to the alternative noninvasive techniques.
Keywords
Brain stimulation, Electrical stimulation, Electrosonic stimulation, Noninvasive brain stimulation, tDCS, TMS
Acknowledgments
We would like to acknowledge Jarrett Rushmore, Uri Eden, Christopher Connor, Virgilio Villacorta, and Antoni Valero-Cabre for the work on the initial electrophysiology studies conducted as part of ( ). That material is based in part upon work supported by the Defense Advanced Research Projects Agency (DARPA) under Contract No. W31P4Q-09-C-0117. The views, opinions, and/or findings expressed are those of the author(s) and should not be interpreted as representing the official views or policies of the Department of Defense or the U.S. Government. Additional work presented herein was also supported in part by Award Number R43NS062530, 1R44NS080632 from the National Institute of Neurological Disorders And Stroke. Any opinions, findings and conclusions or recommendations expressed in this material are those of the authors and do not necessarily reflect the views of the National Institutes of Health.
Introduction
In this chapter, we present a novel form of noninvasive brain stimulation (NIBS), electrosonic stimulation. Electrosonic stimulation combines independently controllable electromagnetic and ultrasonic fields to boost neurostimulation currents via tuned electromechanical coupling in neural tissue. The coupling leads to enhanced stimulation focality, penetration, and targeting control, when compared to their single energy counterpart technologies. We briefly review state-of-the-art electromagnetic and ultrasound NIBS technologies as relevant comparisons to the electrosonic method; then, we present electrosonic stimulation.
Noninvasive Electromagnetic Methods
The two most commonly used electromagnetic-based techniques for NIBS are Transcranial Magnetic Stimulation (TMS) and Transcranial Direct Current Stimulation (tDCS) ( ). TMS and tDCS take advantage of different electromagnetic principles to noninvasively modulate neural activity. TMS employs “focused,” pulsed magnetic fields to induce currents in the brain and influence cortical function. tDCS employs low amplitude, broadly distributed, direct currents, applied via scalp electrodes, to penetrate the skull and enter the brain to influence cortical function. TMS and tES have been used for numerous clinical and experimental applications, including treatment of depression, Parkinson’s Disease (PD), and chronic pain ( ).
Transcranial Magnetic Stimulation
Numerous TMS techniques have been proposed, such as single pulse TMS, repetitive TMS (rTMS), paired pulse stimulation, and theta burst stimulation ( ). TMS uses the principle of electromagnetic induction to focus induced currents in the brain and modulate cortical function. These currents can be of sufficient magnitude to depolarize neurons. Furthermore, when these currents are applied repetitively they can modulate cortical excitability, which can be decreased or increased beyond the actual duration of stimulation ( ).
TMS is thought to actively initiate action potentials (APs) in neurons and/or alter the level of neural excitability during (online) and after (offline) stimulation. Online suprathreshold TMS effects actively initiate APs of stimulated cells, such as those generating phosphenes during perception studies ( ). Offline TMS effects are thought to result from an alteration of the long-term excitability of neural cells and networks following stimulation ( ), although the mechanism is poorly understood ( ).
The distribution of the induced, stimulating, current density is dependent on the location of the TMS coil and the TMS field parameters (i.e., the source characteristics), relative to the targeted tissue (i.e., the field environment). Several factors affect the induced stimulating current density, thus limiting the induced current’s penetration, focality, and targeting control. Although, with TMS, the magnetic fields penetrate the tissues virtually unimpeded, the induced currents distribute based on the boundary conditions between the head tissues ( ). And, it has been shown analytically that TMS currents are always maximum at the cortical surface ( ). Another factor limiting current penetration is represented by coils (where there is a tradeoff between focality and depth ( )). In terms of focality, it has been estimated that areas on the cortical surface at least 100 mm 2 are maximally stimulated on average for M1 stimulation; however it is difficult to assess the effects of the fields in the “subthreshold region” (as they are still significant enough in magnitude to potentially influence cellular response), and when one estimates the penetration through the cortex volumes in excess of 500 mm 3 are stimulated ( ). The control of targeting is also limited in TMS, where oftentimes regions targeted do not correspond to the location of the maximum current density and stimulation, and in fact, many of the clinically used tracking systems which predict the site of stimulation are inaccurate, especially in the presence of pathologies ( ).
TMS techniques are being developed to increase its focality, penetration, and/or usability. developed a coil design where the rate of decay from the surface is attenuated, such that deeper structures can be stimulated (simultaneously with the overlaying cortical surface) without the need of excessive field strengths. Researchers have also investigated the use of conducting shields, placed between the TMS coil and the patients head, to alter and focus the stimulating fields ( ). Finally, groups are developing forms of TMS based on multiple coils ( ), superconducting coils ( ), and ultraportable systems ( ), but there is limited evidence of clinical superiority over the conventional TMS methods.
Transcranial Direct Current Stimulation
Multiple forms of transcranial electrical stimulation (TES) have been proposed, such as tDCS, transcranial alternating current stimulation (tACS), and transcranial random noise stimulation (tRNS) ( ). tDCS is the most common form of TES.
During tDCS, low amplitude, direct currents are applied via scalp electrodes to penetrate the skull and enter the brain. Although the currents applied do not necessarily elicit APs, they can still influence the level of excitability of individual neurons. As with TMS, when tDCS is applied for a sufficient duration, cortical function can be altered beyond the stimulation period ( ). Numerous animal models and cellular studies have explored ( ) and revealed that DC currents can influence spontaneous activity and the evoked response of neurons for hours after just minutes of stimulation in rat preparations. During and following tDCS, neurons are considered “polarized.” Unfortunately what this means at a cellular level is still highly debated, but it has been shown that the probability of spontaneous neural spiking can be altered by “polarizing” a nerve, in a field-to-neural-axis dependent manner. This in turn equates to tDCS’s proposed capability to facilitate and inhibit neural activity (dependent on the polarity of the tDCS source) ( ).
As during TMS, the distribution of the injected stimulating current density distributions are dependent on the source characteristics (i.e., the electrodes and stimulating current), relative to the targeted tissue (i.e., the field environment). tDCS current density distributions are the least focal of the methods reviewed so far and have the same current density and maximum surface limitations as TMS (i.e., constrained to the cortical surface) and limited to no targeting control, when compared to TMS. In terms of focality, it has been estimated that areas on the cortical surface ranging from 300 to 1300 mm 2 are maximally stimulated during M1 stimulation, and when one estimates the penetration through the cortex volumes in excess of 1000s of cubic millimeters are possible ( ). To overcome limitations in focality and targeting control, technologies using arrays of electrodes, such as “High Definition” tDCS (HD-tDCS) and other technologies (e.g., simultaneous EEG monitoring during tDCS as to adjust dosage and parameters) have been proposed ( ). These are explored in greater detail in other chapters in this book.
Nonelectromagnetic Techniques
Numerous nonelectromagnetic techniques have been proposed, including optical ( ), thermal ( ), and acoustic/ultrasonic stimulation. We only briefly discuss acoustic techniques herein, due to the scope of this chapter, and in lieu of other material provided in this publication.
Acoustic Stimulation
Acoustic/ultrasound based brain stimulation has been explored for nearly 60 years ( ) with varying degrees of success. Sonification of nerve cells is thought to initiate an altered neural response, as was discussed by Harvey as early as 1929 ( ). Although work by and and others ( ), exploring low-intensity focused ultrasound in neural slices, animals, and humans have demonstrated encouraging results; it is still not known if lasting acoustic neuromodulatory effects can be harnessed for clinical use and/or if mechanical methods can elicit effects that are comparable to electrical methods ( ), currently used in the clinic.
Currently, the mechanism of pure acoustic-based stimulation is unknown. Everything from heating, altering membrane properties, affecting “stretch” receptors in neural cells, thermodynamic effects, and simply mechanically “banging” open channels has been proposed ( ). General stimulation concepts, related to acoustics, are briefly reviewed in the electrosonic stimulation section.
Electrosonic Stimulation
Electrosonic stimulation is a method for noninvasively stimulating neural tissue with improved focality, penetration, and targeting control, when compared to other noninvasive stimulation methodologies ( ).
During the most common form of electrosonic stimulation, an acoustic field is focused on neural tissue in the region of an applied, subthreshold DC electric field to mechanically perturb the tissue and alter its conductivity (i.e., tissue resistance) and tissue permittivity (i.e., tissue capacitance), relative to the applied electric field ( ). This can generate a displacement current in the tissue that is capable of stimulation (note we will use the term displacement current to refer to any current generated via permittivity perturbations, used synonymously herein with the terms capacitive and polarization current ( )). See Fig. 137.1 . Stimulation can be accomplished in theory, even with low intensity fields, because, at the DC frequency used for electrosonic stimulation, tissue permittivities are considerably elevated due to “alpha dispersion”/counterion effects and capable of sustaining significant displacement currents ( ). Thus, electrosonic stimulation makes use of the inherently high capacitance of biologically active tissues with a simple electromechanical technique, with low field intensities and frequencies, to noninvasively generate new displacement currents in the brain tissue. A review of electromechanical field interactions with nonbiological materials can be found in ( ) and a review of high tissue permittivities, mechanical influences, and dielectric enhancement in ( ). Further theories about microscopic mechanisms (as opposed to macroscopic descriptions) describe the mechanical alteration of local membrane properties in the presence of the electric field ( ), which is beyond the scope of the current chapter.

Electrosonic stimulation, as currently explored in the clinic, has been applied in a subthreshold manner to polarize neurons (i.e., to alter the probability of spontaneous firings of neurons, to inhibit or facilitate neural activity). It is anticipated that the current enhancement occurring in the region of the coupled fields alters the membrane dynamics, similar to when a nerve is “polarized,” but in a more effective manner, due to the displacement current boost. Additionally, electrosonic stimulation can also benefit from the added boost of the mechanical effects on stimulation (i.e., stretch receptor, etc., however this is not yet known).
As with other systems of stimulation, the final stimulatory fields will depend highly on the geometry and properties of the mechanical transducer/electrical electrodes and the properties of targeted tissues. However, the physical principles, which govern this technique do not suffer from the same electromagnetic constraints as the other noninvasive electromagnetic methods ( ). The first advantage is that focusing can be accomplished through the acoustic field ( ) while subthreshold DC currents can be broadly applied to the tissue via scalp surface electrodes, in a manner similar to tDCS ( ). Transcranial ultrasound systems, used for nonstimulatory applications, can obtain foci in the mm 3 range ( ), where other noninvasive stimulation methods are orders of magnitude less focal ( ). The second advantage is that a subcortical focus can also be achieved via the mechanical field with conventional, focused, ultrasound, array systems ( ), while the subthreshold electric field can still penetrate subcortically with large surface electrodes ( ), where other noninvasive electrical techniques are restricted to maximum cortical effects ( ). Additionally, as electrosonic stimulation implements acoustic powers three to five times lower than ablative and other previous methods ( ), there is physiologically negligible skull heating due to standing-wave acoustic energy absorption (as well as negligible tissue heating, cavitation, etc.) ( ). Thus, electrosonic stimulation can be applied for extended durations, well within established ultrasound ( ) and electrical ( ) guidelines, and with greater efficiency than purely electrical or mechanical methods. Furthermore, electrosonic stimulation is electromagnetic in nature, and thus, in theory, could be matched in neural response to other electromagnetic stimulation methods and not suffer from the limitations of purely acoustic techniques. Finally, electrosonic stimulation allows the potential for “real-time” steerable targeting via phased, array, ultrasound systems.
Initial, preclinical, electrosonic stimulation work was completed, comparing the technique with TMS, transcranial ultrasound (TUS), tDCS, and sham techniques ( ). Specifically, electrophysiology experiments were conducted that focused on comparing the combined local visual evoked potentials (VEPs), recorded from a 23-contact electrode, of anesthetized adult cats during and immediately following transcranial stimulations applied to the animal’s primary visual cortex (V1). The results across the animals demonstrated a significant effect in increased magnitude and duration of effects of stimulation when compared to the other forms of stimulation tested; a sample cat is provided in Fig. 137.2 .
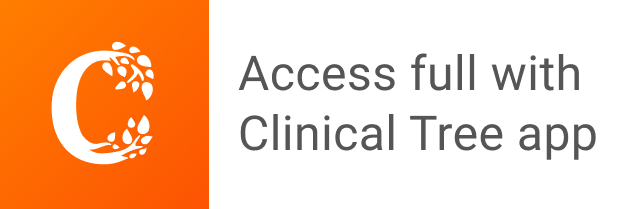