Chapter 11 Pediatric Neuroimaging
Ultrasonography is used extensively for prenatal evaluation and in the neonatal period for evaluation of suspected intraventricular hemorrhage, hydrocephalus, and other developmental anomalies, with CT or MRI used to clarify uncertain findings [van Wezel-Meijler et al., 2010; Soetaert et al., 2009].
During late infancy, childhood, and adolescence, when ultrasound is no longer practical, CT is used in the emergency setting for the evaluation of trauma, stroke, and raised intracranial pressure and for examination after placement of ventricular shunts [Ketonen and Valanne, 2008; Abdelhalim and Alberico, 2009]. In most centers, CT is used as the initial examination for evaluation of closed-head or spine injury because of its superior ability to demonstrate acute hemorrhage or osseous injury. CT is also more commonly used than MRI as the initial method for evaluating children presenting with seizures in the emergency department.
An overview of the strength and weaknesses of pediatric ultrasound, CT, and MRI is provided here. A discussion of the neuroimaging changes associated with normal brain development is beyond the scope of this chapter, but several excellent monographs are available [Barkovich, 2005; Tortori-Donati and Rossi, 2005; Provenzale, 2009].
Cranial Ultrasound
Problematic areas in early diagnosis include the cause of ventriculomegaly, the seriousness of isolated ventriculomegaly, the implications of variations of morphology of Dandy–Walker variants, and the nature of underlying cortex in cases of cerebral dysgenesis [Levine et al., 1997].
Real-time sonography can display echogenic (bright) structures, such as choroid plexus, hemorrhage (Figures 11-1 to 11-5), some tumors, and focal areas of cerebritis; it can also show sonolucent structures, such as cerebrospinal fluid in the ventricles (Figure 11-6), subarachnoid spaces, cysts (Figure 11-7 and Figure 11-8), and cystic lesions (Figure 11-9). Normal brain gives rise to fairly uniform echogenicity.
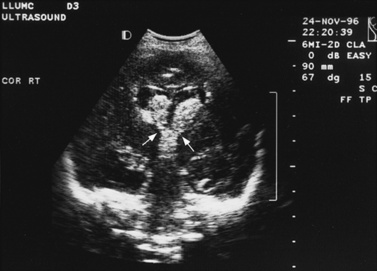
Fig. 11-2 Ultrasound scan of the head of a 1-day-old infant born at 31 weeks’ estimated gestational age.
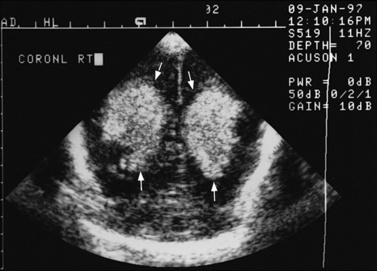
Fig. 11-3 Ultrasound scan of the head of a 3-day-old newborn at 25 weeks’ estimated gestational age.
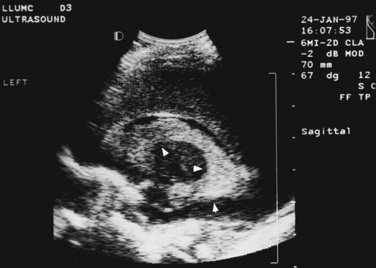
Fig. 11-4 Ultrasound scan of the head of an 8-day-old newborn of 26 weeks’ estimated gestational age.
Detection of fetal CNS anomalies has significantly increased in the last 10–15 years because of the availability of high-resolution ultrasound equipment and the improvement in scanning techniques [Iruretagoyena et al., 2010; Nyberg et al., 2006; Angtuaco et al., 1994]. Transabdominal and endovaginal ultrasound can document early first trimester development and compare it with known embryologic landmarks. The wide array of CNS pathology detected by prenatal sonography includes fetal seizures via abnormal movements [Abrams and Balducci, 1996], holoprosencephaly [Stagiannis et al., 1995], encephalocele [Budorick et al., 1995], schizencephaly, lissencephaly, hydrocephaly (see Figure 11-6) [Holzgreve et al., 1993; Roume et al., 1990], Dandy–Walker malformation (see Figure 11-9) [Cowles et al., 1993], spina bifida [Thiagarajah et al., 1990], and congenital astrocytoma [Heckel et al., 1995].
Postnatally, sonography is helpful in the evaluation of hypoxic-ischemic encephalopathy [van Wezel-Meijler et al., 2010; Kirimi et al., 2002; Anand et al., 1994], including complications of hemorrhage into the germinal matrix, ventricles, or surrounding parenchyma [Sehgal et al., 2009; Müller et al., 2007]. For all infants younger than 30 weeks’ gestation, routine cranial ultrasonography screening should be performed once between 7 and 14 days of age, and should be repeated optimally between 36 and 40 weeks’ postmenstrual age [Ment et al., 2002]. This strategy detects lesions such as intraventricular hemorrhage, which influences clinical care, and those such as periventricular leukomalacia and ventriculomegaly, which provide information about long-term neurodevelopmental outcome [Ment et al., 2002]. Ultrasound can also screen for intrauterine infections and to determine the cause of an enlarging or enlarged head [Yikilmaz and Taylor, 2008; Smith et al., 1998]. Ischemic white matter injury, periventricular leukomalacia (see Figure 11-7 and Figure 11-8), and cystic encephalomalacias can be assessed by ultrasound [Rodriguez et al., 1990], but this approach may not reveal the full extent of injury [Huppi, 2004; Inder et al., 2003; Miller et al., 2003a]. Progression or resolution of intracranial hemorrhage and some of its sequelae, such as hydrocephalus, can be monitored using sonography (see Figure 11-5). Lesions containing cerebrospinal fluid or cerebrospinal fluid-like contents are well delineated because of the echo lucency of water. Examples include ventriculomegaly (see Figure 11-6), porencephaly, Dandy–Walker syndrome (see Figure 11-9), arachnoid cyst, and encephalocele. Vascular malformations, especially aneurysms of the vein of Galen, can be well demonstrated with color Doppler ultrasound. Subtle differences between certain types of lesions, such as differentiation of hydranencephaly from severe hydrocephalus, may not be detected by ultrasound. Major migrational anomalies, such as agyria-pachygyria and lissencephaly, may be delineated by ultrasound; however, smaller heterotopias or other subcortical dysplasias may be overlooked. Ultrasound is helpful in the evaluation of a newborn suspected of having tuberous sclerosis, as it can detect the intracranial hamartomas or subependymal nodules seen in this condition [Tatsuno et al., 1993].
Sonography can be used to screen for myelodysplasia in early infancy, to demonstrate the level of the conus medullaris, and to evaluate newborns suspected of having closed spinal dysraphism (see Chapter 19 for ultrasound examples) [Toma and Rossi, 2001]. It can also reveal the presence or absence of cord pulsations, a sign of tethering, when viewed through the cartilaginous spinal laminae and the infraspinous gaps, which act as sonographic windows. Other dysraphic lesions, such as meningocele, lipoma, hydromyelia, Arnold–Chiari II malformation, diastematomyelia, and lipomyelomeningocele, also can be delineated. Occasionally, sonography is necessary postoperatively to determine the absence of cord pulsations, a finding that suggests cord retethering.
Doppler sonography is used intraoperatively for monitoring resection of arteriovenous malformations during surgery and at the bedside or in the clinic to evaluate arterial patency, direction, and volume of flow and to calculate a resistive index [Lindegaard et al., 1993; Manchola et al., 1993]. Doppler sonography can also be used to evaluate the intracranial circulation during or after extracorporeal membrane oxygenation to detect ischemic or hemorrhagic injury. In patients with subarachnoid hemorrhage, transcranial Doppler ultrasound can be used to detect vasospasm before the patient suffers ischemic neurologic deficit or infarct [Topceuoglu et al., 2002]. It is a standard technique to determine the absence of cerebral blood flow in patients for whom the diagnosis of brain death is suspected.
Computed Tomography
CT has been available since the 1970s for clinical use in children. Ionizing radiation due to x-ray CT is effectively restricted to the immediate body part of interest by the tight collimation used to create the thin fan beam used for scanning. Considerable technical developments have taken place with CT in the past three decades. Several generations of scanners are available, including the helical CT introduced in the early 1990s and the multidetector or multislice scanner. This availability has resulted in an increased number of computed tomographic scans of children, increasing the potential for inappropriate use and excessive radiation dosing [Frush et al., 2003]. Some data indicate that low-dose radiation, similar to the amount received with CT, may be associated with a significant risk of cancer, especially in young children. Children have a longer lifetime in which to manifest radiation-related cancer, and the cancer risk from CT is cumulative over a lifetime. Each computed tomographic examination contributes to the lifetime exposure. It is necessary to limit radiation from CT in children and follow the ALARA (“as low as reasonably achievable”) principle. Several strategies can be followed toward this goal, including performing only necessary examinations, considering alternative imaging modalities such as ultrasonography or MRI when possible, limiting the region of coverage, and adjusting individual imaging parameters, depending on indication, region imaged, and size of the child [Frush et al., 2003; Singh et al., 2009; Strauss et al., 2010].
Abnormalities can be characterized with CT as having low density, isodensity, or high density in relation to the brain. Lesions that appear lower in density include edema, necrosis, infarction (Figure 11-10), neoplasms, leukodystrophies, inflammation, and cysts. Loss of gray–white matter differentiation may be seen with diffuse brain edema after hypoxic-ischemic injury or a demyelinating process. Fat-containing lesions usually appear less dense than water or of mixed density, as in patients with a teratodermoid type of tumor. Air appears as the lowest density, and can be seen with pneumocephalus after trauma or surgery. Lesions isodense to normal tissues are difficult to recognize unless there are changes that demonstrate displacement or replacement of normal anatomic structures or intravenous contrast material that helps to separate the lesion from normal structures. High-density lesions identified on CT usually indicate hemorrhage or the presence of calcium (Figure 11-11). Pathologic intracranial calcifications can be seen with congenital infections, neurocysticercosis, intracranial tumors, tuberous sclerosis, Sturge–Weber syndrome, neurofibromatosis, Cockayne’s syndrome, hypoparathyroidism, arteriovenous malformations, vein of Galen malformations, encephalomalacia, cerebral infarction, or the sequelae of perinatal asphyxia [Erdem et al., 1994]. Hypercellular neoplasms with high nuclear to cytoplasmic ratios (e.g., medulloblastomas, other primitive neuroectodermal tumors, germinomas, lymphomas) may also appear as high-density lesions on CT examination. In young children, CT usually requires sedation and occasionally enhancement using intravenous contrast material. CT myelography and cisternography can be accomplished with intrathecal contrast material introduced into the subarachnoid space. Computed tomographic imaging can be used for precise localization during needle-biopsy procedures or volumetric resection of pediatric brain tumors [Kelly, 1991]. In the evaluation of neonatal disorders, a non-contrast-enhanced computed tomographic examination after ultrasound is helpful in the evaluation of acute hemorrhage (Figure 11-12 and Figure 11-13), developmental anomalies (Figure 11-14 and Figure 11-15), acute trauma (Figure 11-16 and Figure 11-17), suspected infarction (Figure 11-10A), and hydrocephalus, or to demonstrate atrophy and mineralization.
In childhood neurodegenerative diseases, CT may reveal decreased attenuation in the basal ganglia and cerebral white matter or focal or generalized cerebral atrophy [Mirowitz et al., 1991]. Non-contrast-enhanced CT can be helpful in evaluating neurocutaneous syndromes to detect calcifications as in tuberous sclerosis (see Figure 11-15) and for the evaluation of the patient with acute onset of seizures. After closed-head injury, CT without contrast material is usually optimal for evaluation of the skull, intracranial hemorrhage, orbits, sinuses, facial or temporal bones, and osseous spine for fractures or other types of injuries. Evaluation of these regions is of particular importance in the assessment of young children who are victims of nonaccidental trauma. Non-contrast-enhanced CT of the head can rapidly evaluate possible skull fracture and intracranial hemorrhage [Dashti et al., 1999]. Imaging patterns of intracranial hemorrhage in children younger than 3 years have been reported to predict whether the injury was intentional [Wells et al., 2002]. These patterns include subdural hemorrhage located over the cerebral convexities, hemorrhage within the interhemispheric subdural space, hygroma (i.e., nonhemic subdural fluid) with intracranial hemorrhage, and absence of a skull fracture with intracranial hemorrhage [Wells et al., 2002]. If there is a high-risk mechanism of injury, a normal neurologic examination and maintenance of consciousness do not preclude significant intracranial injury in a pediatric trauma patient. A liberal policy of computed tomographic scanning is warranted for such patients [Simon et al., 2001].
Contrast-enhanced CT is helpful in the evaluation of suspected or known vascular malformation, neoplasms (see Figure 11-11B), abscesses, and empyemas. In infants and children with chronic subdural hematomas, contrast enhancement may reveal an enhancing membrane. Contrast-enhanced CT also can be used to differentiate infarction from neoplasm or abscess. Follow-up computed tomographic studies may help distinguish developing infarction from neoplasm. Contrast-only CT may be obtained for serial evaluations of patients with neoplasms, vascular malformations, or suppurative collections. In patients with suspected metastases or seeding of neoplasms, contrast-only CT scan may be sufficient. However, in all the clinical situations previously described, MRI is considered more definitive.
Magnetic Resonance Imaging
On T2-weighted (T2W) images, tissues or structures with long T2 relaxation times, such as cerebrospinal fluid, edema, many tumors, extracellular methemoglobin, infarcts, and multiple sclerosis plaques, are bright, whereas tissues or substances such as muscle, cortical bone, deoxyhemoglobin, and hemosiderin are dark as a result of short T2 relaxation times (see Figure 11-20, Figure 11-23, and Figure 11-25 below). Inversion recovery sequences are typically used to nullify or eliminate signal from a particular tissue. For example, fluid-attenuated inversion recovery (FLAIR) sequences nullify signal from fluids, causing CSF to show up dark rather than bright on T2W images. This sequence is used to increase conspicuity of lesions that are bright on T2 (multiple sclerosis plaques, infarcts) and located near the ventricles, and is particularly useful for identifying subacute subdural hemorrhage. Another sequence called short tau inversion recovery (STIR) nullifies signal from fat and can be used to identify lesions in fatty areas such as the orbits or neck. GRE sequences are routinely used to provide T2*W contrast in images to identify hemorrhage. Since the increased use of higher field strength clinical imaging magnets at 3T, GRE is being used routinely to provide T1W contrast since GRE sequences have less energy deposition than SE sequences and can be performed faster to provide imaging during breath hold. Routinely, T1- and T2-weighted images in various planes are obtained and followed by T1W images with contrast, if needed. The most commonly used contrast agent for MRI contains gadolinium, which shortens the T1 relaxation time of tissues that take up gadolinium contrast agent and thus produce a bright or hyperintense signal on T1W images.
MRI should be used as the primary imaging modality for screening patients in whom neurologic disease is suspected because it is generally the most sensitive and specific of the imaging techniques. MRI is the only modality that provides accurate assessment of brain myelination [Harbord et al., 1990]. Contrast-enhanced MRI is also more sensitive than contrast-enhanced CT for detection of encephalitis [Koelfen et al., 1996], brain abscess (Figure 11-18), ventriculitis, or small foci of leptomeningeal involvement. MRI is often better than CT in demonstrating traumatic injuries, particularly diffuse axonal shearing injury [Mittle et al., 1994], post-traumatic olfactory injury [Yousem et al., 1996], brainstem contusion, subacute hemorrhage, encephalomalacia, and gliosis. Certain pathologies – including migrational anomalies such as gray-matter heterotopias, closed-lip schizencephaly, lissencephaly, pachygyria (Figure 11-19), and hemimegalencephaly, and neurocutaneous disorders such as neurofibromatosis [Es et al., 1996] – that are not seen well on CT are better demonstrated with MRI. In children with severe head injury who are comatose, the presence of traumatic brainstem lesions seen on MRI has a high correlation with outcome. CT fails to demonstrate these lesions [Woischneck et al., 2003].
MRI is useful in the evaluation of patients with movement disorders, such as Wilson’s disease [King et al., 1996; Magalhaes et al., 1994], because it demonstrates abnormal T2 signal in the basal ganglia, especially the outer rim of the putamen and in the substantia nigra; in juvenile Huntington’s disease, it reveals atrophy of the caudate nuclei and increased T2 signal in atrophic caudate nuclei [Ho et al., 1995]. In pantothenate kinase deficiency (formerly designated as Hallervorden–Spatz syndrome), MRI demonstrates areas of symmetric low-signal intensity in T2-weighted images with high signal intensity in the anteromedial aspect of the globus pallidus, the so-called eye of the tiger sign [Ou et al., 1994; Savoiardo et al., 1993]. MRI shows multifocal cortical infarctions and the presence of lactate peaks in proton MRS in mitochondrial disorders, such as MELAS syndrome (i.e., mitochondrial encephalopathy with lactic acidosis and strokelike episodes) [Castillo et al., 1995; Barkovich et al., 1993; Kim et al., 1996; Wycliffe and Skeen, 1997]. In other mitochondrial disorders, such as MERRF syndrome (i.e., myoclonus, epilepsy, and red ragged fibers), Kearns–Sayre syndrome, Leigh’s syndrome, Alpers’ disease, and Menkes’ disease, symmetric white matter T2 hyperintensities with involvement of deep cerebral nuclei are observed on MRI. Patients with Menkes’ disease demonstrate rapidly progressive atrophy accompanied by large subdural hematomas [Barkovich et al., 1993].
For focal (Figure 11-20) and diffuse white matter disease, T2-weighted MRI is more sensitive than CT in demonstrating lesions. MRI should preferentially be used to evaluate children with acute disseminated encephalomyelitis (Figure 11-21) [Lee et al., 1996], human immunodeficiency virus (HIV) encephalitis, sickle cell disease, vasculitis such as systemic lupus erythematosus, Lyme disease [Oksi et al., 1996], progressive multifocal leukoencephalopathy, and multiple sclerosis [Guilhoto et al., 1995]. Recognizable patterns of white matter involvement can be seen with MRI in certain inherited leukodystrophies. For example, early, diffuse involvement of the peripheral subcortical white matter is seen in Pelizaeus–Merzbacher disease [Andre et al., 1990], Canavan’s disease, and Alexander’s disease. In Canavan’s disease, patients have macrocephaly, and on proton MRS, a larger than normal N-acetyl-aspartate peak is produced. In Alexander’s disease, there is predilection for T2 lengthening in frontal white matter and enhancement after contrast administration. A predilection for occipital white matter involvement is seen with adrenoleukodystrophy. Follow-up MRI examinations have been used for patients with adrenoleukodystrophy to monitor progression of disease symptoms [Loes et al., 1994]. In globoid cell leukodystrophy (i.e., Krabbe’s disease), T2 hyperintensity can be seen in the cerebellum and deep cerebral white matter [Sasaki et al., 1991], whereas the thalami and basal ganglia may be hypointense [Osborn and Tong, 1996].
MRI can demonstrate mesial temporal sclerosis (Figure 11-22) in patients with epilepsy [Kuzniecky et al., 1996], and can reveal small tumors in the aqueduct, sella turcica, or brainstem (Figure 11-23) even when the CT is normal. Children with mesial temporal sclerosis may have dual pathology with mild to moderate cortical dysplasia and with hippocampal sclerosis that can be visualized with MRI [Mohamed et al., 2001]. Continuing technical advances are reported using higher field strengths (3T) and/or multiple channel coils that provide higher signal to noise to obtain high-resolution images of the brain capable of detecting lesions not previously identified with MRI, including hippocampal dysplasia, hippocampal atrophy, and dual pathology with cortical dysplasia. These high-resolution MRI scans are improving the diagnosis of patients who are candidates for surgical treatment for refractory epilepsy [Goyal et al., 2004]. MRI is also the preferred procedure when children present with symptoms and signs that suggest a CNS tumor (Figure 11-24; see also Figure 11-11 and Figure 11-23). In the evaluation of pediatric head and neck lesions, MRI provides valuable additional information concerning lesions that are difficult to resolve with CT, and it is essential for assessment of possible intracranial extension of disease [Jones and Koch, 1999].
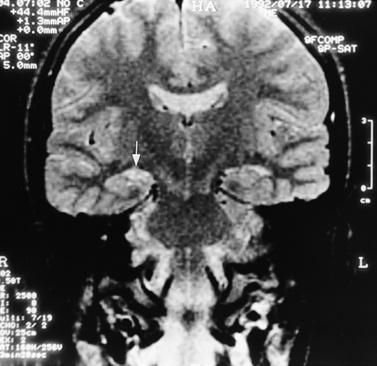
Fig. 11-22 T2-weighted, coronal image through the hippocampus in a child with temporal lobe seizures.
For vascular and hemorrhagic lesions, MRI is more specific than CT or ultrasound. MRA has added a new dimension to the evaluation of pediatric cerebrovascular disease. This noninvasive imaging technique can be used to evaluate vascular malformations, vaso-occlusive disease (see Figure 11-13C and Figure 11-20B), and vascular neoplasms. MRA may demonstrate occlusions or stenoses of the supraclinoid internal carotid arteries, the proximal anterior and middle cerebral arteries, collateral vessels, and parenchymal lesions in moyamoya disease [Yamada et al., 1995], as well as proximal large-vessel stenotic lesions in children with sickle cell disease [Wiznitzer et al., 1990].
MRI may detect silent infarctions in children with Kawasaki’s disease [Fujiwara et al., 1992] or sickle cell disease. In cases of childhood migraine and in sickle cell disease (see Figure 11-20), MRI may demonstrate small white matter and basal ganglia infarctions not seen on CT. MRI is more specific than CT in the identification and staging of hemorrhage and clot formation as a function of the evolution of hemoglobin breakdown products (Figure 11-25) and can differentiate arterial from venous occlusive disease. Sinovenous thrombosis in children is a serious condition that can result in neurologic impairment or death in approximately half the patients [deVeber and Andrew, 2001]. The presence of a venous infarct or dural sinus thrombosis seen with MR venography predicts a poor outcome. In evaluating intracranial hemorrhage caused by angiographically occult lesions such as cavernous angiomas (see Figure 11-25), MRI is the procedure of choice. MRI can help to differentiate between various infantile extra-axial fluid collections and may distinguish effusions and hygromas from subdural hematomas, as in child abuse. Special MRI sequences (FLAIR) can be used to differentiate cerebrospinal fluid-containing lesions (e.g., arachnoid cysts) from other lesions (e.g., epidermoid tumors) that are often difficult to visualize on CT. Sagittal MRI images have better resolution than ultrasound and also cannot be obtained using CT. MRI is excellent in delineating midline abnormalities including lesions in the sella, aqueduct, foramen magnum, and pineal region (Figure 11-26). Absence of T1 hyperintensity in the posterior pituitary on a sagittal MR image is diagnostic of central diabetes insipidus in children [Maghnie et al., 2000]. For evaluation of hypopituitarism in children, MRI of the head is critical [Geffner, 2002]. Hydrocephalus in children, unexplained by ultrasound and CT, may be evaluated satisfactorily with an MRI examination (Figure 11-27). Likewise, in patients with CNS tumors and cerebrospinal fluid seeding, MRI with gadolinium enhancement is more sensitive than a CT examination. MRI is also being used for planning surgery, radiotherapy, and chemotherapy, as well as for treatment follow-up. Contrast enhancement adds to the usefulness of MRI in these settings.
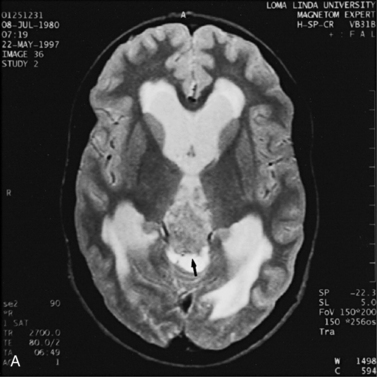
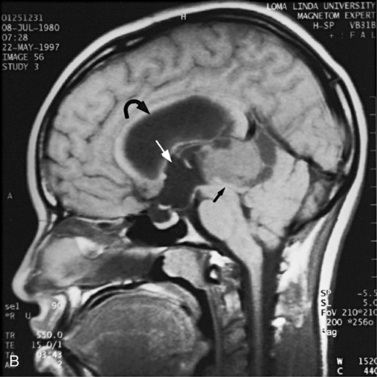
Fig. 11-26 A 16-year-old female with 2 months of blurred vision, who was found to have bilateral papilledema.
Magnetic Resonance Spectroscopy
MRS is a clinically useful, noninvasive tool for identifying the biochemical state of the CNS [Novotny et al., 1998; Panigrahy et al., 2010]. It can provide information about certain metabolites in normal and pathologic conditions [Ross and Michaelis, 1994]. Certain atomic nuclei such as 1H (protons) are magnetic, and when exposed to a strong magnetic field, they align in a particular orientation until equilibrium is reached [Prichard, 1993]. If the nuclei are then excited by a radiofrequency pulse at their resonant frequency, they produce a detectable signal during relaxation back to equilibrium. During relaxation, because of their local chemical environment, each proton produces a signal at a slightly different frequency, called a chemical shift. After a Fourier transform analysis, the plots of the resulting nuclear magnetic resonance spectra appear as peaks of signal intensity versus signal frequency or chemical shift. Peaks at a particular chemical shift represent signal from protons of a particular metabolite or biochemical compound while the area underneath the peak represents the relative concentration of the metabolite.
MRI uses the strong signals from 1H nuclei of water and their spatial location to reconstruct anatomic images. In contrast, proton MRS is interested in the much weaker signals from nonwater protons to obtain quantitative information about biologic molecules within tissue. Special techniques, such as chemical shift selective saturation (CHESS), are used to suppress the overwhelming signal produced because of the large concentration of water. Small concentrations of brain metabolites can be detected using acquisition sequences, such as stimulated-echo acquisition mode and point-resolved spectroscopy. The principles underlying this spectral editing are quite complex and are discussed in several reviews [Bolinger and Insko, 1996; Novotny et al., 1998; Ross and Michaelis, 1994].
Initial studies in children used phosphorus (31P) MRS to investigate spectral changes seen after neonatal asphyxia [Martin et al., 1996]. Reduced phosphocreatine to inorganic phosphate (PCr/Pi) ratios suggested that impairment of energy metabolism occurred late rather than early after asphyxia, and this ratio correlated with poor neurologic outcome, as did the presence of lactate in other studies [Groenendaal et al., 1994; Martin et al., 1996].
Spectral Metabolites Using Proton Magnetic Resonance Spectroscopy
Proton MRS (1H-MRS) is being used to investigate a wide range of neurologic disorders. Unlike 31P MRS, 1H-MRS can be obtained using the same coils as imaging. Metabolites measured with 1H-MRS include N-acetyl-aspartate (NAA), a neuronal marker; creatine (Cre) composed of phosphocreatine and creatine, which are bioenergetic metabolites; choline-containing compounds (Cho), including free choline and phosphoryl and glycerophosphoryl choline that are released during membrane disruption; lactate (Lac), which accumulates in response to tissue damage or anaerobic glycolysis; and other metabolites only seen when acquired with short echo time sequences, such as the neurotransmitters glutamate and immediately formed glutamine (Glx) and myoinositol (mI or Ins), an osmolyte and astrocyte marker [Prichard, 1991; Sappey et al., 1992; Tunggal et al., 1990]. During normal maturation of the brain, NAA, Cre, and Glx levels increase, and Cho and Ins levels decrease [Kreis et al., 1993; Ross et al., 1992] (Figure 11-28). The newborn brain has high water content, and the high Cho and Ins levels decline as myelination progresses [Kreis et al., 1993]. It is not uncommon to detect small lactate peaks in spectra from normal newborn brain, but if lactate levels are high or appear after the neonatal period, it may indicate possible hypoxic-ischemic injury [Holshouser et al., 1997], a metabolic disorder, or infection.
Diseases Studied with Proton Magnetic Resonance Spectroscopy
Characteristic proton spectra in adults have been described in stroke, hepatic encephalopathy, dementia, diabetic ketoacidosis, tumor, multiple sclerosis, acquired immunodeficiency syndrome-related leukodystrophies, and trauma [Ross and Michaelis, 1994]. In children, abnormalities on 1H-MRS have been observed in a variety of metabolic [Chabrol et al., 1995; Kruse et al., 1994] and mitochondrial encephalopathies [Abe et al., 2004], brain tumors [Arle et al., 1997; Byrd et al., 1996; Sutton et al., 1992; Taylor et al., 1998; Warren et al., 2000], and certain neurodegenerative conditions [van der Knapp et al., 1992; Wang et al., 1996]. Since metabolite levels change with brain maturation, metabolite levels and ratios must be compared to normal age-matched control values acquired with the same sequence and parameters at the same field strength for proper interpretation of the results.
Proton MRS has been used after acute traumatic and nontraumatic injuries. Adult patients with severe closed-head injuries or stroke with long-term neurologic sequelae demonstrate elevated Lac peaks, reduced NAA peaks, and reduced NAA/Cre ratios [Choe et al., 1995; Matthews et al., 1995]. After closed-head injury in children, Lac has been reported in regions of contusion and infarction but not in areas of diffuse axonal injury [Sutton et al., 1995]; Lac elevation is more common in children with nonaccidental trauma [Holshouser et al., 1997; Aaen et al., 2010]. Other researchers have found that reduced occipital NAA/Cre ratios or the presence of cerebral Lac correlated with poor outcomes [Ashwal et al., 2000; Shu et al., 1997]. Increased NAA/Cho and reduced Cho/Cre ratios correlate with impaired neuropsychologic functioning after traumatic brain injury [Brenner et al., 2003; Babikian et al., 2006; Hunter et al., 2005]. Spectral sampling in areas of brain that did not appear to be visibly injured has shown altered metabolite ratios that suggested definite injury. Later studies have examined the role of mI, a marker for post-traumatic astrogliosis or disturbed osmotic regulation, using quantitative short echo time MRS, and have found that occipital gray matter mI levels are increased after traumatic brain injury and correlate with poor outcomes [Ashwal et al., 2004b]. The Glx and Cho levels are elevated for all traumatic brain injury patients compared with control subjects, whereas NAA was not [Ashwal et al., 2004a]. The time interval before MRS acquisition after injury and location of sample within the brain might affect findings. Overall, these studies suggest the strong potential for using MRS to provide accurate estimates of long-term neurologic and neuropsychologic function after traumatic brain injury in infants and children. More recently, a longitudinal study using more advanced MR imaging techniques such as susceptibility weighted imaging (SWI) and diffusion tensor imaging (DTI) (discussed in later sections) along with MRS has shown these techniques to be capable of detecting post-traumatic hemorrhagic lesions and the corresponding abnormalities on DTI, as well as metabolite abnormalities on MR spectroscopic imaging (MRSI) (Figure 11-29).
MRS with diffusion-weighted imaging (DWI) has been used to evaluate children with stroke and hypoxic-ischemic injury. Confirmation of the death of tissue is provided on proton MRS by a rise in Lac from anaerobic glycolysis, and a loss of NAA from neuronal death [Zimmerman, 2000]. The combination of MRI and MRS can provide prognostic information in children after near-drowning [Dubowitz et al., 1998]. MRI and MRS have been found particularly useful in the evaluation of neonates with perinatal encephalopathy. Reduced NAA-derived metabolite ratios, elevated Cho or Ins, as well as abnormalities on DWI, have been shown to reflect the severity of injury and to correlate with long-term developmental and neurologic outcomes [Bartha et al., 2004; Cady et al., 1997; Robertson et al., 2001; Zarifi et al., 2002; Miller et al., 2005]. Patients with elevated brain Lac levels and decreased NAA/Cre ratios have been significantly more likely to have a poor outcome compared with age-matched control subjects (Figure 11-30) [Holshouser et al., 1997].
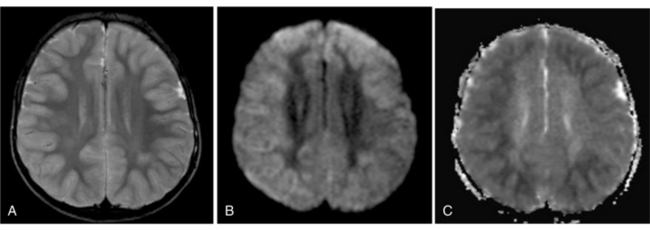
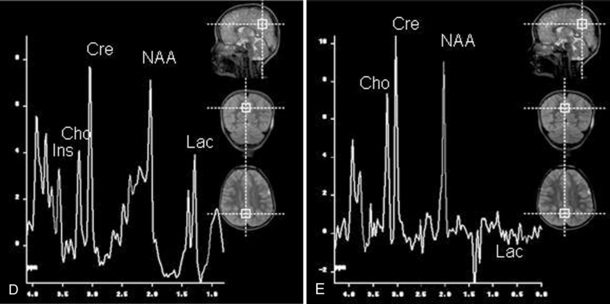
Fig. 11-30 A 30-month-old male after cardiopulmonary arrest following a near-drowning incident.
A, T2-weighted, axial MRI. Diffuse T2 hyperintensities are seen along the cortical margin of both cerebral hemispheres involving the gray matter, which is consistent with diffuse cytotoxic edema from hypoxic-ischemic insult. B, Diffusion-weighted image (b = 1000) shows diffuse abnormal increased signal in the cortical gray matter and subcortical region of both cerebral hemispheres corresponding to the areas of T2 abnormality. C, Apparent diffusion coefficient (ADC) map shows relative low signal in the subcortical region of both cerebral hemispheres extending into the cortical margin. D, Single-voxel proton MR spectrum (TR = 3000 msec, TE = 20 msec, stimulated-echo acquisition mode [STEAM]) from occipital gray matter obtained 4 days after injury reveals a prominent lactate (Lac) peak and a markedly reduced N-acetyl-aspartate/creatine ratio (NAA/Cr = 0.78) compared with age-matched controls (NAA/Cr = 1.5 ± 0.14) (see Figure 11-28A–D). E, Proton MR spectrum (TR = 3000 msec, TE = 144 msec, point-resolved spectroscopy [PRESS]) of the same voxel area obtained immediately after the spectrum depicted in B also reveals a prominent lactate peak and a markedly reduced NAA peak, both of which indicate a poor prognosis. This patient had minimal neurologic response and was withdrawn from life-support 7 days after incident.
Proton MRS combined with MRI is useful in screening patients for metabolic and mitochondrial disorders based on the detection of increased cerebral lactate or the presence of other elevated metabolite peaks [Ashwal et al., 1997; Barkovich et al., 1993; Chabrol et al., 1995; Cross et al., 1993; Matthews et al., 1993]. Abnormalities have been reported in patients with glutaric aciduria type 2 [Shevell et al., 1995]; pyruvate dehydrogenase deficiency [Cross et al., 1994]; Leigh’s syndrome [Krageloh et al., 1993]; X-linked adrenoleukodystrophy [Kruse et al., 1994], MELAS [Abe et al., 2004; Kaufmann et al., 2004], and a variety of other conditions. In phenylketonuria, elevated phenylalanine levels can be seen with MRS of the brain [Osborn and Tong, 1996]. In Canavan’s disease, MRS shows marked elevation of the NAA peaks [Osborn and Tong, 1996]. In addition to an elevated NAA peak, patients with Canavan’s disease have abnormally increased NAA/Cre and NAA/Cho ratios [Barker et al., 1992]. In Leigh’s disease, as in other mitochondrial or metabolic disorders, MRS reveals an abnormally high Lac peak and a decreased NAA peak in the basal ganglia and occipital gray matter [Osborn and Tong, 1996] (Figure 11-31). In some children with metabolic diseases, Lac is present during the acute illness, and with improvement, serial spectra can demonstrate its resolution [Kreis et al., 1995]. Elevated cerebral Lac has also been detected when blood Lac levels have been normal in symptomatic patients [Krageloh et al., 1993]. In children with global developmental delay, proton MRS has also been useful in detecting creatine deficiency associated with disorders of creatine synthesis or transport [Newmeyer et al., 2005].
< div class='tao-gold-member'>
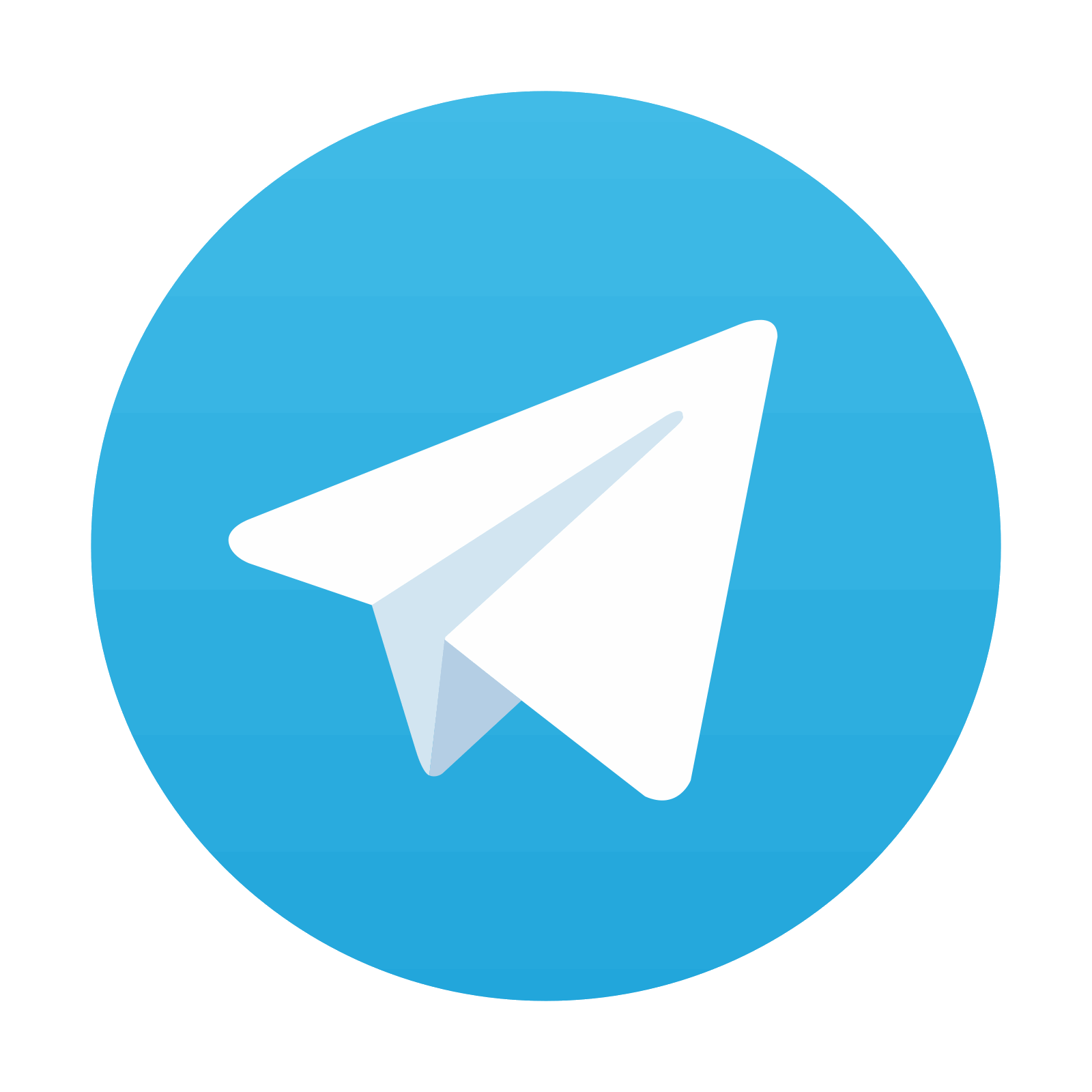
Stay updated, free articles. Join our Telegram channel
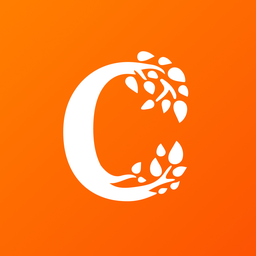
Full access? Get Clinical Tree
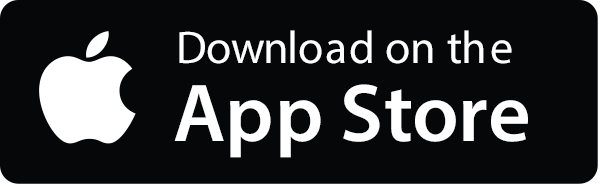
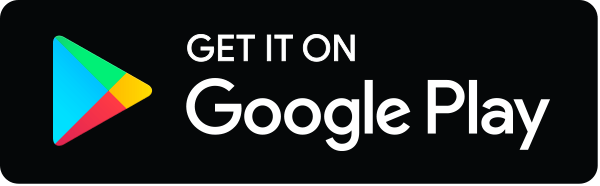