Introduction
Individuals with Down syndrome (DS) are at extremely high risk for Alzheimer’s disease (AD), with the vast majority of adults with DS developing AD neuropathology by 40 years of age (Chapters 1 and 2). This includes the presence of amyloid plaques, neurofibrillary tangles, and granulovacuolar degeneration [1–4]. Similarly, most individuals with DS will exhibit indications of clinical decline (resulting in a diagnosis of dementia) by 60 years of age [5, 6] (Chapter 15). The etiology of AD in DS is primarily due to the overproduction of amyloid-beta (Aβ) that appears to begin early in life. Aβ overproduction is caused by the presence of three copies of chromosome 21, each containing one copy of the Amyloid Precursor Protein (APP) gene [7–9]. Yet, factors in addition to overexpression of APP likely contribute to the wide variation in age of onset of AD in the DS population, including lifestyle (e.g., Chapter 12), gender, genetics (Chapter 10), and comorbid medical conditions [3, 10, 11] (Chapter 11).
Based upon data from both cross-sectional and longitudinal studies, the earliest signs of AD neuropathology in the non-DS population occur approximately 20 years prior to the appearance of clinical symptoms [12]. Referred to as the “cascade hypothesis,” early neuropathological signs start with the aggregation of soluble Aβ42 in the brain into insoluble plaques, as evidenced by reductions in concentrations of Aβ42 in the cerebrospinal fluid (CSF), followed by amyloid deposition. Subsequent increases in CSF tau and phosphorylated tau occur, which are indicative of neuronal injury. The formation of neurofibrillary tangles follows, along with changes in brain structure, such as decreased hippocampal volume and glucose hypometabolism [13]. It is at this point that the earliest clinical symptoms can be detected, typically in episodic memory [14, 15]. While it is hypothesized that the pathophysiological features of AD in adults with DS are similar to that of late-onset AD (neurotypical individuals > 65 years of age), the course of the AD disease process in the DS population is only partially understood. There is well-documented evidence of histologically detectable diffuse Aβ plaques in adolescents and young adults with DS, consistent with early amyloid pathology observed in neurotypical adults at the preclinical and prodromal stages of sporadic AD [16, 17]. The vast majority of adults with DS will evidence significant cortical Aβ deposition by the early 1940s, along with documented changes in brain structure (e.g., enlarged lateral ventricles and reduced hippocampal volume) [18–20]. Changes in functioning and cognition tend to first appear by the mid-to-late 40s, with the mean age of diagnosis of AD in DS adults occurring in the early to mid-fifties, depending upon the study [21, 22]. Survival following a diagnosis of dementia appears to be shorter in comparison to late-onset AD [21].
Understanding the cascade of pathologic changes of AD in DS and its modifiers has obvious implications for the development of treatment and preventative strategies. There are other populations, such as individuals with autosomal dominant forms of AD (APP, Presenilin-1 and Presenilin-2 mutations), that are known to experience accelerated amyloid pathology and early AD onset. However, the high prevalence of DS (over 250,000 people living with DS in the United States) versus relatively few individuals with autosomal dominant AD mutations provides a much larger target group for the study of AD biomarkers.
The definitive diagnosis of AD and the characterization of primary AD pathology and other contributing pathological features of disease presentation and course were previously only possible with direct histopathological examination of brain tissue. With the advent of advanced molecular, structural, and functional neuroimaging techniques, AD pathology can now be reflected in vivo. This encompasses a wide range of biomarkers that have been divided into three categories, each representing a different underlying pathophysiology [23]. Referred to as the AT(N) classification system, it comprises: (1) “A”—Aβ biomarkers (e.g., amyloid PET, CSF Aβ42); (2) “T”—tau biomarkers (e.g., tau PET, CSF p-tau); and (3) “N”—biomarkers representing neurodegeneration or neuronal injury (e.g., CSF t-tau, FDG- PET, structural MRI). Using this classification system, each category can be dichotomized as positive (+) or negative (−), resulting in scores such as A +/T +/N − or A +/T −/N −. While this system has been shown to have promising utility in classifying individuals with AD [24], it has not yet been used widely among individuals with DS.
PET imaging is recognized as a key component of the AT(N) classification system, providing important biomarker measurements in all three classification categories. In this chapter, we first provide a brief history of the development of PET imaging and radiotracers AD investigation, followed by more detailed information on the three primary types of PET radiotracers that have been used in AD and DS research. Finally, we provide an overview of the research utilizing PET imaging in the DS population.
Utility of pet imaging for studying ad pathology
PET neuroimaging is uniquely suited for detecting the presence of AD-related pathology. Through the use of radiolabeled drugs, typically small molecules of several hundred daltons, it is possible to assay the presence of AD-related pathology, such as beta amyloid and tauopathies, in concentrations in the nanomolar range. Widespread use of PET scanners and accompanying cyclotrons for the production of chemically relevant β + emitting radionuclides (e.g., 11C and 18F) have provided the necessary methodologies for extending PET brain imaging into targeted AD-related pathology. Some of the earliest applications of clinical research in the field of AD PET imaging were directed toward detecting function changes in cerebral perfusion (using [15O] water), oxygen utilization ([15O]O2), and glucose utilization (using [18F]fluorodeoxyglucose—FDG) in neurodegenerative disease [25–27]. Because glucose is the brain’s primary energy source, FDG has proven to be highly informative for demonstrating the decline in glucose metabolism (hypometabolism) and its relationship with decline in cognitive function. Further, FDG imaging has been used to characterize the signature brain regions of AD-related decline in the parietal, frontal, and temporal cortices.
Current AD research utilizing the power of PET imaging is focused primarily on detecting early stage, i.e., presymptomatic changes in brain function and neurochemistry related to the presence of AD-related neuropathology. The detection of submicromolar levels of proteinopathies (e.g., amyloid beta and tau) has identified the radiotracer properties required for accurate and sensitive detection. Favorable in vivo radiotracer properties include optimal blood-brain barrier penetrance, high ligand-receptor affinity with reversible and saturable binding, minimal radiolabeled metabolites crossing BBB, and high target biochemical specificity. This last issue of target specificity is of critical importance within the context of binding to the beta-sheet structure of the cross-β conformations of amyloids (see Mathis et al. [28] for review of radioligand considerations for amyloid beta and tau).
The in vivo imaging characteristics of the PET radiotracers also have a major role in determining the sensitivity of the outcome measures for providing a quantitative index for the levels of AD neuropathology present. Ideally, the PET scan would yield a direct measure of the quantity of pathology, such as the concentration of amyloid beta binding sites (Bavail of Aβ) in the brain, to provide a comparison with clinical symptoms and neuropathological assay. The most commonly used index of receptor concentration is “binding potential” (BP), defined as available receptor concentration (Bavail) scaled either to the radiotracer concentration in the arterial plasma (BPF, BPP) or to a PET measured brain region representing a reference region of both free and nonspecifically bound (referred to as nondisplaceable) radiotracer (BPND) [29]. The calculation of BP requires acquisition of a PET time series, starting with the intravenous injection of radiotracer until a specified period of time to allow for the radiotracer to bind to the target and clear from the plasma and tissue at a steady rate, typically requiring > 1 h of scanning.
The acquisition of a lengthy PET scan required for BPND measurement can be a challenge for many populations, particularly in the elderly, both with and without dementia. The use of PET scanning procedures with truncated scan times has been developed to minimize participant burden. Although these shortened procedures forego the ability to directly measure BP as an outcome of neuropathology, metrics have been validated that serve as a satisfactory proxy to BP. The standardized uptake value ratio (SUVR) represents the PET measured concentration from a single time window (e.g., 90–110 min) from a target region divided by a reference region containing negligible pathology, ideally acquired at a time of radiotracer pseudo-equilibrium between the specifically bound and free spaces. The reference region often represents gray matter brain tissue. The nondisplacement signal, containing both unbound (i.e., free) and nonspecifically bound radiotracer, is similar to the target region (e.g., SUV [precuneus c]/SUV [gray matter cerebellum]) and effectively cancels out this nondisplaceable component in the ratio. However, reference regions containing white matter are frequently used to provide increased PET signal from a larger sampling volume, despite having different nondisplaceable properties.
Although binding potential is proportional to the available concentration of receptor sites, it is also dependent on the affinity of the radioligand for the receptor, with each PET radiotracer having unique ligand-protein affinities for amyloid beta and tau. Because of this, it is not possible to directly compare the calculated BP from two different PET radiotracers targeted the same receptor site. This restriction also applies to a cross-radiotracer comparison of the SUVR outcome, due to both distinct ligand-target affinities and nonspecific binding characteristics of the radiotracers. The “centiloid” metric was created to normalize the SUVR measures from the various amyloid radiotracers (e.g., PiB, florbetapir, florbetabin, flutemetamol) across a common range [30]. The linear rescaling of the SUVR for each radiotracer to a range of 0 (young healthy controls) to 100 (mild-moderate AD) permits a direct comparison of amyloid load across all radiotracers. Region-of-interest templates have been developed for each amyloid radiotracer to ensure the use of robust and rigorous methods when using centiloid units. A second method for standardizing PET amyloid outcomes across radiotracers is the use of the amyloid load index [31], which also scales the measured amyloid levels from 0% to 100% based on the capacity of brain tissue to hold amyloid beta. A brain template has been created specific to the DS population for the calculation of amyloid load for the Alzheimer’s Biomarker Consortium-Down Syndrome (ABC-DS study) [32, 33]. The use of these normalized outcomes also facilitates establishing cut-points to dichotomize the continuous variables into classifications of “positive” and “negative” amyloid status.
The contributions of PET imaging and the use of the PET outcomes for the study of AD-related pathology in DS are summarized in the following sections. While FDG imaging for neurodegeneration represents the biomarker for the last stage of the AT(N) framework, it is presented first in this review that provide historical context for demonstrating the important role PET imaging has played in deepening our understanding of the progression of AD-related pathology.
FDG PET
One of the earliest uses of PET imaging of the brain involved FDG-PET (F-18 labeled fluorodeoxyglucose), which measures an index of regional cerebral metabolic rate for glucose (rCMRGl). Glucose is the brain’s primary energy source and its uptake parallels synaptic activity. Most forms of dementia result in a global decline in glucose metabolism (hypometabolism), although there are often regional differences in the pattern of decline. For example, in one of the earlier studies of PET imaging in AD, Messa et al. [34] successfully used FDG-PET to distinguish between AD and vascular dementia. Today, frontotemporal dementia (FTD) is typically distinguished from AD via FDG PET, due to its distinctive and contrasting pattern of hypometabolism. A study by Foster et al. [35] demonstrated that FTD results in hypometabolism in the anterior cingulate, anterior temporal and frontal regions in contrast to the posterior cingulate and posterior temporoparietal cortex. Individuals with AD exhibit the opposite pattern with FDG PET.
Clinically, FDG PET can be useful when an individual’s history is ambiguous, when the clinical presentation is atypical, or when symptoms and features are shared by more than one disorder [36]. While the exact pattern of decreases in rCMRGl may differ across patients with AD, the first region to be involved is typically the posterior cingulate gyrus. Subsequent affected areas include the association cortex of the parietal and posterior temporal lobes. Finally, the prefrontal cortex and most of the brain are affected [35]. However, some regions are relatively spared, including the caudate, putamen, and thalamus, but remain hypometabolic in comparison to nonaffected individuals [37]; the cerebellum and brain stem remain relatively unaffected [38]. Current thinking is that glucose hypometabolism begins approximately 10 years prior to the diagnosis of AD in both late-onset AD and autosomal dominant forms of AD [39, 40].
There have been only a small set of published studies involving the use of FDG PET in adults with DS. One of the earliest studies compared four groups: middle-aged healthy adults with DS (n = 17), middle aged age-matched controls (n = 12), late-onset older adults (non-DS) with AD (n = 10), and a group of older age-matched controls (n = 12) [41]. Both the DS and AD groups were found to have lower rCMRGl than the age-matched controls in the posterior cingulate and fusiform gyrus. However, the individuals with DS had increased rCMRGl in the inferior temporal/entorhinal cortex compared to both AD and matched controls, which was interpreted as a possible compensatory response during early disease progression. This same group of investigators subsequently reported the results of a four-year follow-up study, now including structural MRI in a group of 19 adults with DS, 17 of whom were initially evaluated in the earlier study [19]. Consistent with their prior findings, the strongest correlations were found between increased rCMRGl and decreased gray matter volume in parts of the temporal cortex (including the parahippocampus/hippocampus, thalamus, caudate, and frontal lobe), adding additional support for a compensatory brain response in adults with DS in the preclinical stages of AD.
More recently, Sabbagh and colleagues [18] compared FDG PET outcomes across a group of five adults with DS and dementia, 12 healthy adults with DS, and 9 normal controls. The posterior cingulate rCMRGl in those with DS and dementia was significantly reduced in comparison to both the healthy DS group and normal controls. In addition, the healthy adults with DS evidenced significantly lower rCMRGl than normal controls. Another recent effort, conducted by Rafii et al. [42], called the Down Syndrome Biomarker Initiative (DSBI), examined amyloid PET, FDG PET, and T1 MRI in 12 nondemented adults with DS (ages 30–60 years). Results indicated an inverse relationship between amyloid burden and rCMRGl. The DSBI study also reported on patterns of FDG metabolism in DS that were distinct from patterns associated to AD [43].
There have been several additional papers in the past 2–3 years studying FDG patterns in DS, typically in combination with other imaging modalities. For example, a 2018 study [44] compared amyloid PET, T1 MRI, and FDG PET findings in a group of 24 healthy adults with DS (age 39 ± 7 years). Both rCMRGl hypometabolism and decreased gray matter volume were noted in individuals who were amyloid positive versus those who were amyloid negative. Fortea and colleagues [22] shared cross-sectional data from a cohort of 388 adults with DS for whom a wide range of potential AD biomarkers were obtained. These included cerebral spinal fluid (CSF Aβ1-42, CSF p-tau, CSF NfL), APOE, Plasma Aβ1-42, NfL and Total tau, and amyloid PET. In addition, 197 individuals underwent FDG PET scans. Analysis of the FDG PET data found glucose hypometabolism to be located in the temporoparietal, precuneus-posterior cingulate, and frontal areas among individuals who were displaying symptoms of dementia. Based upon this cross-sectional data, it was determined that decreases in rCMRGl first occur 12–13 years before expected AD symptom onset among adults with DS. Finally, the ABC-DS study recently reported glucose hypometabolism throughout the signature AD-related brain regions in a group of adults with DS (N = 90; 38.0 ± 8.3 years.) and significant negative associations between amyloid and FDG, and these measures were associated with cognition and metabolic connectivity for distinguishing cases of MCI-DS/AD from cognitively stable DS [45].
Special interest in glucose metabolism has also focused on the striatal region, where the earliest evidence of amyloid deposition has been documented among both the DS and autosomal dominant populations [40, 46]. The ABC-DS findings showed no evidence of reduced metabolism in the striatum despite revealing the highest levels of amyloid burden. Similar findings were reported for the autosomal dominant population, with no evidence of glucose hypometabolism in this region of elevated amyloid burden [40].
Amyloid PET
The first amyloid PET radiotracer, called Pittsburgh Compound B (PiB), was radiolabeled with the positron emitting radionuclide, 11C, and scanned in human subjects in 2002. [11C]PiB was shown to detect and quantify the presence of fibralized amyloid in living individuals [47]. Since the initial development of [11C]PiB, a number of related, 18F labeled PET ligands have been developed to take advantage of the longer-lived radiolabel (18F:t1/2 = 110 min; 11C:t1/2 = 20.3 min) which affords the opportunity for production and distribution at a regional radiopharmacy. Examples of 18F labeled radiopharmaceuticals that have gained FDA approval for Aβ imaging include [18F]florbetapir (Amyvid), florbetaben (Neuraceq), and flutemetamol (Vizamyl). It should be noted that if an imaging center has the necessary resources (including an onsite cyclotron and equipment for radiopharmaceutical manufacturing) for in-house production, there are several distinct advantages to using [11C]PiB for Aβ imaging. An example involves PET experiments requiring two scans, such as paired amyloid/tau studies. When using 11C radiotracers for the first scan, a second scan (using 18F) can be initiated after a 2-hour decay period, thus completing the PET imaging session in a single day. Whereas the use of two F-18 radiotracers (e.g., [18F]amyvid →[18F]flortaucipir) would require two separate days for scanning. This strategy is used as some sites for the ABC-DS study to eliminate an overnight stay for the participants with DS traveling extensive distances. Another advantage is the reduced radiation dose exposure to study participants when using the shorter half-life 11C radiolabel, with [11C]PiB scans (555 MBq injection) resulting in approximately 30% of the radiation dose received from the 18F Aβ radiotracers (using 370 MBq injection).
We now have close to two decades of amyloid PET research providing both cross-sectional data and longitudinal data in AD. Regional cutoffs have been developed for individual tracers, allowing for the determination of amyloid-positive versus amyloid negative status. Findings based on research in late-onset AD indicate that approximately 30% of cognitively normal older adults and 60% of older adults with mild cognitive impairment (MCI) are amyloid PET positive [48]. Conversely, special populations, such as autosomal dominant AD and DS, will be almost entirely amyloid positive years prior to the presence of clinical symptoms of AD. There has been a considerable, international research effort to study the autosomal dominant AD population, for which there is now extensive cross-sectional and longitudinal data across a wide range of potential AD biomarkers. For example, a multicenter, cross-sectional study comparing 88 mutation carriers with 40 noncarriers found that none of the noncarriers had detectable amyloid deposition (i.e. all well below the cutoff for amyloid positivity). Conversely, mutation carriers had significant amyloid deposition in the precuneus up to 15 years before their expected onset of clinical symptoms (based upon the age that the affected individual’s parent had developed symptoms, called the “ESO”). Higher PiB SUVR values were observed the closer an individual was to their ESO [39]. A subsequent paper summarized the results of a set of longitudinal follow-up visits for 142 autosomal dominant AD carriers and 75 noncarriers [49]. It was found that, once started, cortical Aβ deposition appears to occur in a relatively linear fashion with a rate of increase similar to sporadic AD. While the amyloid pathology (both cortical and from CSF) appears to trigger subsequent change in other AD biomarkers and measures of cognition, this relationship may not be linear. Instead, once a certain threshold of Aβ is attained, CSF Aβ and tau peptides seem to plateau while other measures, such as hippocampal volume and cognition, display a slower change, followed by a rapid acceleration of abnormality.
The first reported uses of amyloid PET in the DS population appeared in 2011 and 2012 [46, 50, 51]. For example, Handen and colleagues [46] found that among seven healthy adults with DS (ranging in age from 20 to 44), only the two oldest individuals (ages 38 and 44) evidenced significant amyloid deposition with PiB PET. Similar to that observed in presenilin-1 mutation (ADAD) carriers [52], a striatal-predominant amyloid retention pattern was noted (see Fig. 1). Subsequent studies using PiB PET confirmed that PiB retention was highest in the striatum compared to other cortical regions of interest. In fact, the majority of individuals with DS who were determined to be “amyloid positive” evidenced amyloid positivity in the striatum. In some cases, the striatum was the only region of interest that was found to be amyloid positive [53–55]. Jennings et al. [56] assessed amyloid load via [18F]florbetaben PET imaging in a group of 39 adults with DS (46.3 ± 4.7 years). Age and [18F]florbetaben SUVR were significantly correlated, with 90% of individuals > 50 years of age deemed to be amyloid positive, 53% of those individuals aged 45–49, and 7% of those aged 40–45 years. Annus et al. [53] examined amyloid deposition patterns using PiB PET in 49 adults with DS, ages 25–65 years. The earliest abnormal PiB deposition was noted at 39 years of age, initially in the striatum, followed by rostral prefrontal-cingulo-parietal regions, then caudal frontal, rostral temporal, primary sensorimotor and occipital regions. Using some of the participants from the Annus et al. [53] study (along with 30 typically developing adults as a control group), Cole et al. [57] utilized machine learning to predict brain age from structural MRI findings. In addition, the controls and 46 adults with DS underwent PiB PET and cognitive assessment. Both increased amyloid deposition and poorer cognitive performance was found to be associated with age-related brain atrophy. Finally, nonstriatal subcortical areas were affected (e.g., parahippocampal cortex, thalamus, amygdala). As reviewed by Abrahamson et al. [58], this proposed hierarchy did not perfectly match cross-sectional findings from neuropathology studies in DS, in which neocortical regions (including the temporal cortex) were impacted earlier than subcortical regions (including the striatum).

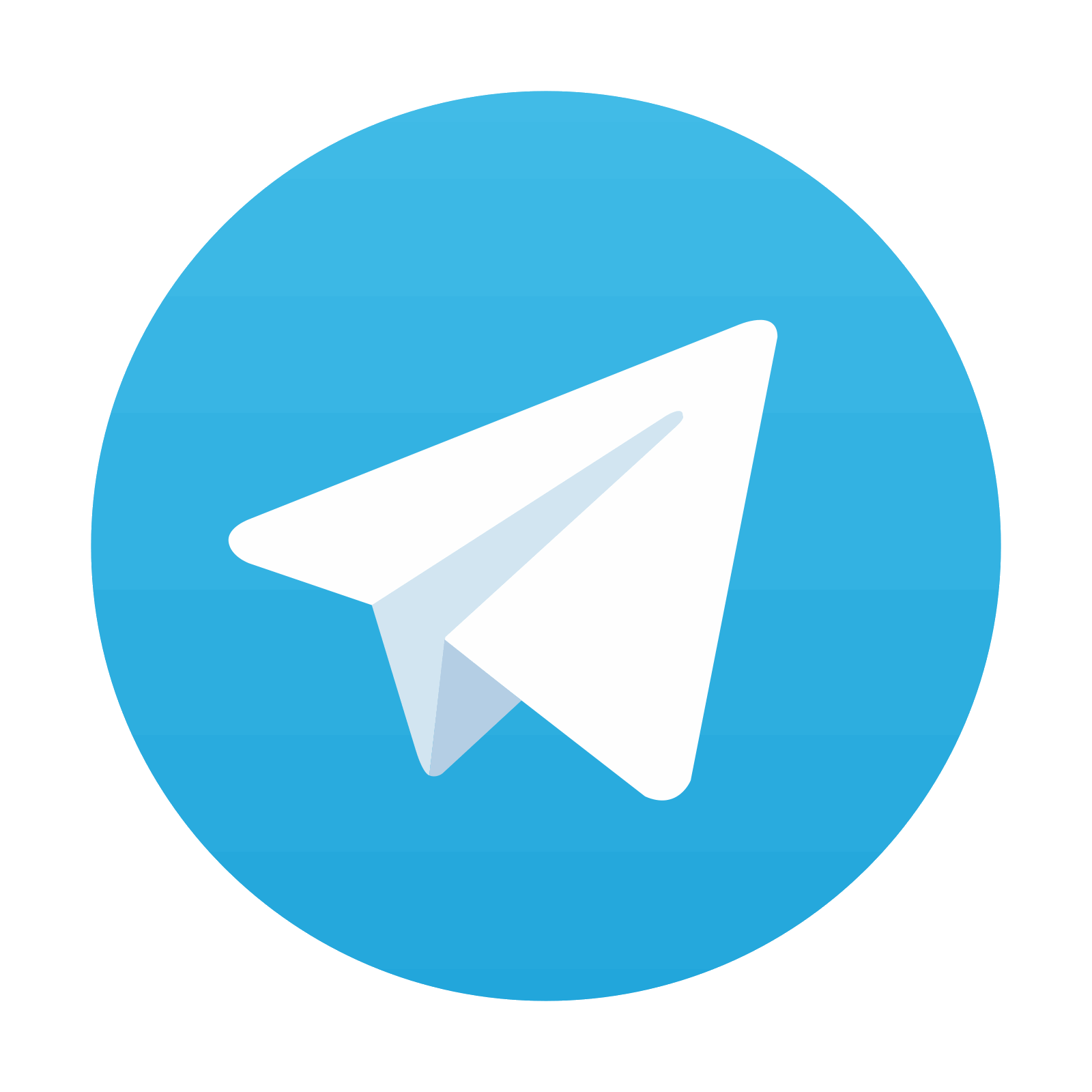
Stay updated, free articles. Join our Telegram channel
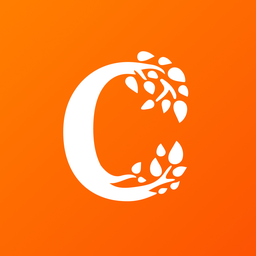
Full access? Get Clinical Tree
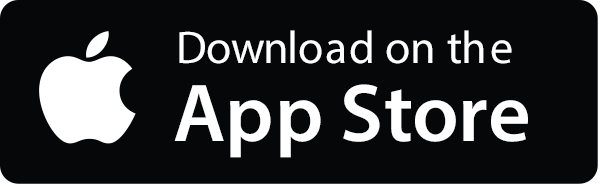
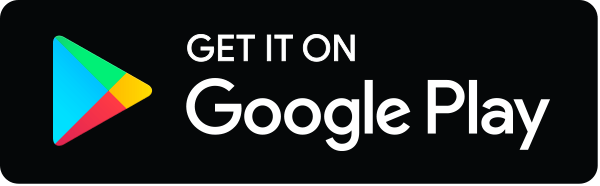