Table 41.1 Physiologic Changes during Wakefulness, NREM Sleep, and REM Sleep | ||||||||||||||||||||||||||||||||||||||||||||||||||||||||||||||||||||||||||||||||||||||||||||
---|---|---|---|---|---|---|---|---|---|---|---|---|---|---|---|---|---|---|---|---|---|---|---|---|---|---|---|---|---|---|---|---|---|---|---|---|---|---|---|---|---|---|---|---|---|---|---|---|---|---|---|---|---|---|---|---|---|---|---|---|---|---|---|---|---|---|---|---|---|---|---|---|---|---|---|---|---|---|---|---|---|---|---|---|---|---|---|---|---|---|---|---|
|
fruit flies (Drosophila) and mammals has been made (26). Dysfunction of the circadian rhythm results in several important sleep disorders including delayed sleep phase syndrome and advanced sleep phase syndrome.
mattress, a closet, and night stand. The sleeping room should be quiet and sound attenuated so that noises from outside the sleep laboratory and technologist’s area do not disturb the sleeping patient. An intercommunication system for contact with the patient is essential. Toilet facilities should preferably be attached to the bedroom and a shower should also be readily available.
quantification of blood oxygen levels are necessary and accomplished using pulse oximetry. Endoesophageal (intrathoracic) pressure recording is also sometimes used in the detection of partial obstructions or upper airway resistance that can exist without actual apneas or hypopneas. The nasal pressure transducer (NPT) is recommended for the measurement of subtle reductions in airflow and can also be used in the identification of absent airflow, characteristic of apnea.
uses a Stow-Severinghaus glass/ceramic electrochemical sensor, with a small heater unit to facilitate blood flow. Transcutaneous monitoring of CO2 tension is more reliable than transcutaneous measurement of O2 because CO2 diffuses better through the skin.
Table 41.2 Filter Settings for PSG Recordings | ||||||||||||||||||||||||||||||
---|---|---|---|---|---|---|---|---|---|---|---|---|---|---|---|---|---|---|---|---|---|---|---|---|---|---|---|---|---|---|
|
associated with increasing delta activity. Stage N3 is scored when 20% or greater of an epoch is delta activity (Fig. 41.6). For the purposes of sleep scoring, delta activity is defined as activity of 0.5 to 2 Hz that is at least 75 µV in amplitude when measured over the frontal or central regions. Sleep spindles may persist into stage N3, but eye movements are usually absent. Axial EMG is usually lower than stage N2 and may approach that seen in stage R.
![]() Figure 41.3 The limited PSG montage illustrates alpha activity (well seen after eye closure) consistent with wakefulness or stage W (30-second epoch, chin = submental EMG). |
![]() Figure 41.5 The limited PSG montage illustrates sleep spindles (sinusoidal activity in top two channels) and broad vertex waves consistent with stage N2 (30-second epoch, chin = submental EMG). |
clinical diagnosis made in the clinic based primarily on the history and does not require PSG evaluation. PLMS is a diagnosis made on the PSG as most patients do not have daytime symptoms and observer history is not usually helpful. Most patients with RLS will also have PLMS, but PLMS most commonly occur in the absence of restless legs symptoms. Periodic limb movements in wakefulness (PLMW) are not part of the recently published AASM scoring guidelines (12). However, several investigators have suggested the utility of PLMW in assessing patients with RLS. The scoring criteria are exactly the same as those outlined above for the scoring of PLMS. These investigators have also proposed a SIT test (suggested immobilization test) with associated quantification of PLMW as a diagnostic test for patients with RLS (41).
syndrome consistent with OSA but without scorable apneic events. With the use of NPT and the associated identification of scorable hypopneas, it is much rarer to have a clinical suspicion of unrecognized respiratory events contributing to a patient’s symptoms. However, the NPT is frequently overly sensitive in that it demonstrates no airflow (an apnea) while the thermal sensor continues to demonstrate obvious airflow. As such, the NPT is not to be used for determination of apneic events, which are to be scored off of the thermal sensor recording.
![]() Figure 41.10 An arousal from stage R with appearance of >3 seconds of faster frequencies accompanied by the appearance of EMG in the axial (chin) EMG channel (30-second epoch). |
NPT signal decreases by >30% for at least 10 seconds.
There is an accompanying oxygen desaturation of 4% or more.
NPT signal decreases by >50% for at least 10 seconds.
There is an accompanying oxygen desaturation of 3% or more or the event is accompanied by an EEG arousal within 3 seconds of the event.
Score sinus tachycardia for sustained sinus heart rate >90 beats per minute.
Score sinus bradycardia for sustained sinus heart rate <40 beats per minute.
Score asystole for cardiac pauses >3 seconds.
Score wide complex tachycardia for a rhythm of at least three consecutive beats at a rate greater than 100 beats per minute and a QRS duration of > 120 msec.
Score narrow complex tachycardia for a rhythm of at least three consecutive beats at a rate >100 beats per minute and a QRS duration of < 120 msec.
Score atrial fibrillation if there is an irregularly irregular ventricular rhythm associated with replacement of consistent P waves with variable rapid oscillations.
Other significant arrhythmias (such as heart block) should be reported if quality of the single lead is sufficient for accurate identification.
gold standard for looking at sleep macrostructure. CAP presents another way of looking at NREM sleep within those sleep stages.
The recurring high-amplitude EEG activity (phase A of the period)
The intervening background EEG activity (phase B of the period)
The period or cycle that is the sum of phase A and phase B
![]() Figure 41.16 The three phase A subtypes. The dotted line indicates the fast low-amplitude portion of phase A. EEG derivation as in Figure 41.15. (From Terzano et al. Atlas, rules, and recording techniques for the scoring of cyclic alternating pattern (CAP) in human sleep. Sleep Med. 2001; 2:537-553, with permission.) |
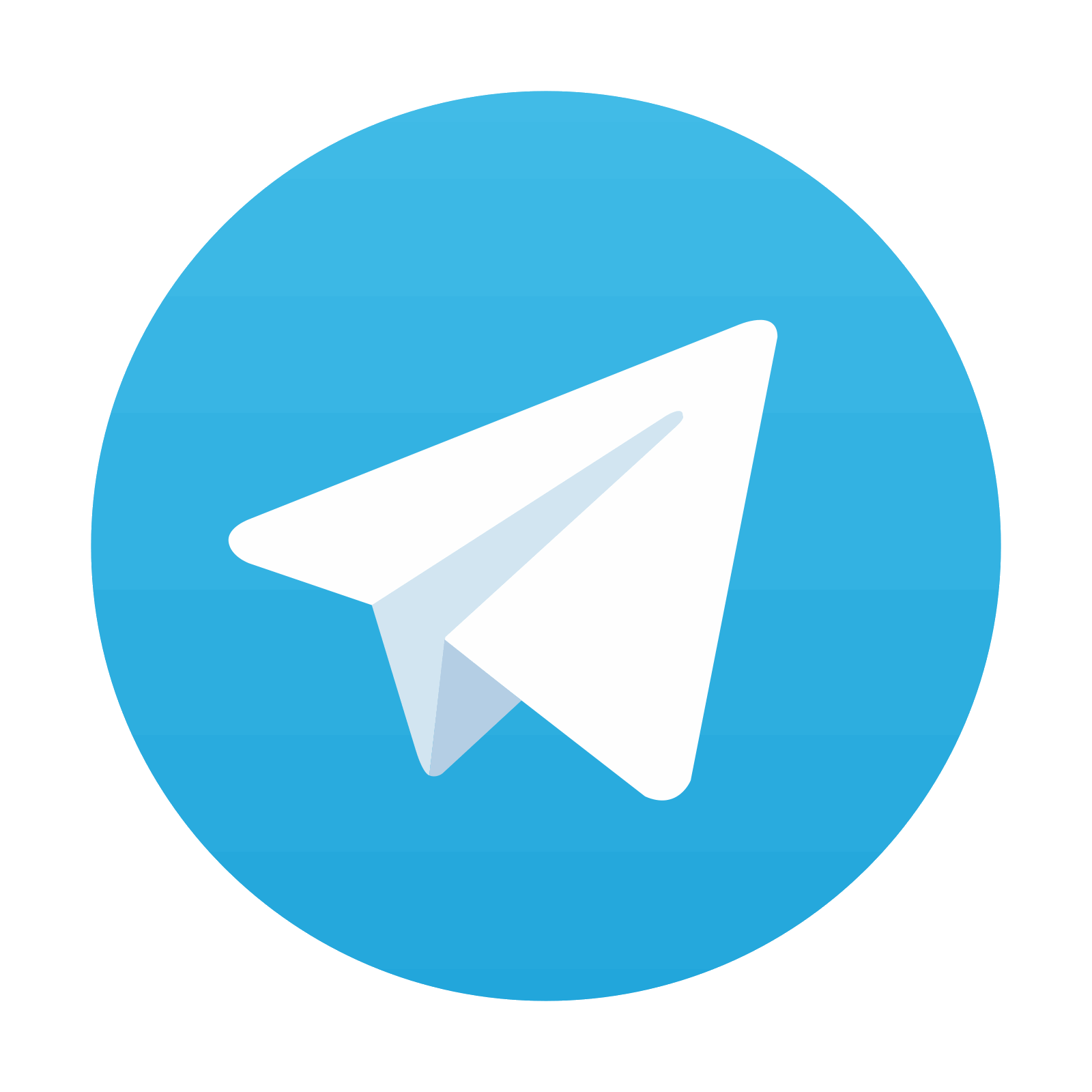
Stay updated, free articles. Join our Telegram channel
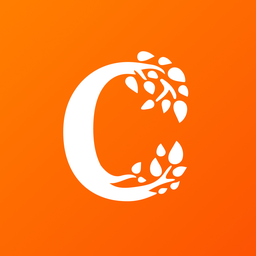
Full access? Get Clinical Tree
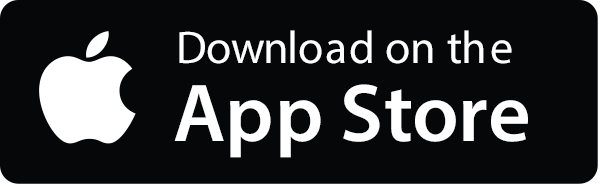
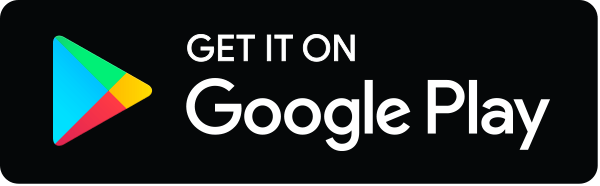