Positron Emission Tomography and Single-Photon Emission Computed Tomography
William Charles Kreisl
INTRODUCTION
Molecular imaging is used in the clinical practice of nuclear medicine and in medical research to better understand the biochemical processes that underlie human disease. Two examples of molecular imaging are positron emission tomography (PET) and single-photon emission computed tomography (SPECT). In both PET and SPECT, patients are administered a radioactive compound with a pharmacokinetic behavior that targets a molecular pathway related to the pathology of a certain disease.
PET and SPECT allow highly sensitive and selective measurement of specific biologic changes in the human body. Agents are administered in amounts too small to cause pharmacologic effects (“trace” doses) so as to avoid perturbation of the biochemical pathway being studied. Many radioactive compounds used in PET and SPECT reversibly bind to a target protein via ligand-receptor kinetics. In these cases, the radioactive ligand is referred to as a radioligand. However, some radioactive compounds used for PET or SPECT do not bind a target protein. For example, [15O]H2O is used to measure perfusion, as the uptake of this compound reflects the flow of blood into a given tissue. A [15O]H2O PET scan therefore does not measure the binding of water to any receptor, but rather it traces the flow of blood to different tissues (Fig. 22.1). In this case, the term radiotracer is more appropriate. A broader term that applies to all compounds used with PET or SPECT is radiopharmaceutical, reflecting that the radioactive compounds used in molecular imaging are given the same regulatory considerations as drugs.
BASIC PRINCIPLES
Both PET and SPECT employ the physical phenomenon of radioactive decay, which occurs in radionuclides that are unstable due to incompatible number of protons and neutrons or excess energy.
In PET imaging, radionuclides are proton rich and as a result undergo decay and emission of a positron. An example of a radionuclide is carbon-11 (11C), which has six protons and five neutrons. Unstable due to the greater number of protons than neutrons, 11C undergoes a decay event in which a proton is converted to a neutron and the nucleus emits a positron and a neutrino. The positron will then zigzag around the vicinity of the decay event, losing energy as it collides with electrons of neighboring atoms, until it comes almost to rest and combines with an electron. Because a positron and electron are of equal mass but opposite charge, this event results in an annihilation encounter, with two 511 keV gamma rays (photons) emitted at about 180 degrees from each other. Other examples of positron-emitting radionuclides used for PET are 18F, 15O, and 13N.
In SPECT imaging, radionuclides exist in a metastable state and decay from their excited state to ground state, resulting in emission of a single photon. The gamma-emitting radionuclides 99mTc and 123I are commonly used for SPECT.
RADIOPHARMACEUTICALS AND SELECTION OF RADIONUCLIDES
Radionuclides are combined with a pharmaceutical through a series of synthetic steps to create a radiopharmaceutical. Radiopharmaceuticals are administered either intravenously or orally and once in the biologic system, they interact with different molecules based on the kinetic properties of the pharmaceutical to which the radionuclide is attached. The radionuclide will then undergo nuclear decay as described earlier, ultimately resulting in the emission of two photons in the case of PET and a single photon in the case of SPECT. The radiation exposure to the patient is a function of the radionuclide used and the amount of radiopharmaceutical administered. Radionuclides with longer half-lives (e.g., 18F, t½ = 110 minutes) result in larger exposure than those with shorter half-lives (e.g., 11C, t½ = 20.5 minutes). However, the advantage of radiopharmaceuticals with long half-lives is that they may be synthesized offsite and delivered to medical facilities, providing greater availability for clinical use. Use of radiopharmaceuticals with short half-lives is limited to facilities capable of onsite synthesis.
DETECTION, ACQUISITION, AND DISPLAY OF SIGNAL
When radiopharmaceutical decay events occur inside a PET or SPECT scanner, crystals in a scintillation detector absorb the photons and then emit pulses of light that are then amplified, sorted, and registered as a count. The sum of these counts creates a tomographic map of all the decay events that occur within the view of the scanner. The result is an image that represents the different densities of radioactivity throughout the tissues captured by the
scanner. The basis of PET and SPECT imaging is that the majority of decay events occur in proximity to where the radiopharmaceutical is acting on the molecular target of interest. The sensitivity of PET and SPECT is a function of the fraction of emitted photons that contribute to the total image. In PET, coincidence detection of the pair of photons that are emitted per decay event allows greater efficiency than in SPECT, which captures only the single photons that approach the detector at certain angles. Therefore, PET is generally more sensitive than SPECT and is more frequently used for quantitative imaging, although absolute quantitation has been reliably attained with SPECT.
scanner. The basis of PET and SPECT imaging is that the majority of decay events occur in proximity to where the radiopharmaceutical is acting on the molecular target of interest. The sensitivity of PET and SPECT is a function of the fraction of emitted photons that contribute to the total image. In PET, coincidence detection of the pair of photons that are emitted per decay event allows greater efficiency than in SPECT, which captures only the single photons that approach the detector at certain angles. Therefore, PET is generally more sensitive than SPECT and is more frequently used for quantitative imaging, although absolute quantitation has been reliably attained with SPECT.
PET and SPECT images reflect only the amount of radioactivity detected by the scanner. The scanner cannot distinguish between sources of radioactivity (which radionuclide or which radiopharmaceutical was administered). Therefore, sufficient time (at least six half-lives of the radionuclide) must pass between consecutive scans on the same subject; otherwise, the second scan will be contaminated from residual signal from the first. In addition, once injected, radiopharmaceuticals are broken down by enzymes in the liver and other tissues (including brain in some instances) leading to the generation of radiolabeled metabolites. If these metabolites are generated in the brain or cross from the blood into the brain, they will contribute to the total signal in the resulting images, potentially confounding accurate quantification. For this reason, using radiopharmaceuticals that do not generate radiometabolites that cross the blood-brain barrier is beneficial. Another problem is cross-selectivity and nonspecific binding. If a radiopharmaceutical binds more than one receptor in the brain, the resulting image will overestimate the density of the intended target. As radiopharmaceuticals must be lipophilic to cross the blood-brain barrier, they often bind nonspecifically to lipids throughout the brain. Both specific binding to the target protein and nonspecific binding contribute to the signal seen on the resulting image. However, only the specific binding component represents the density of the target protein. Therefore, a high ratio of specific to nonspecific binding is favorable for reliable PET and SPECT measurements.
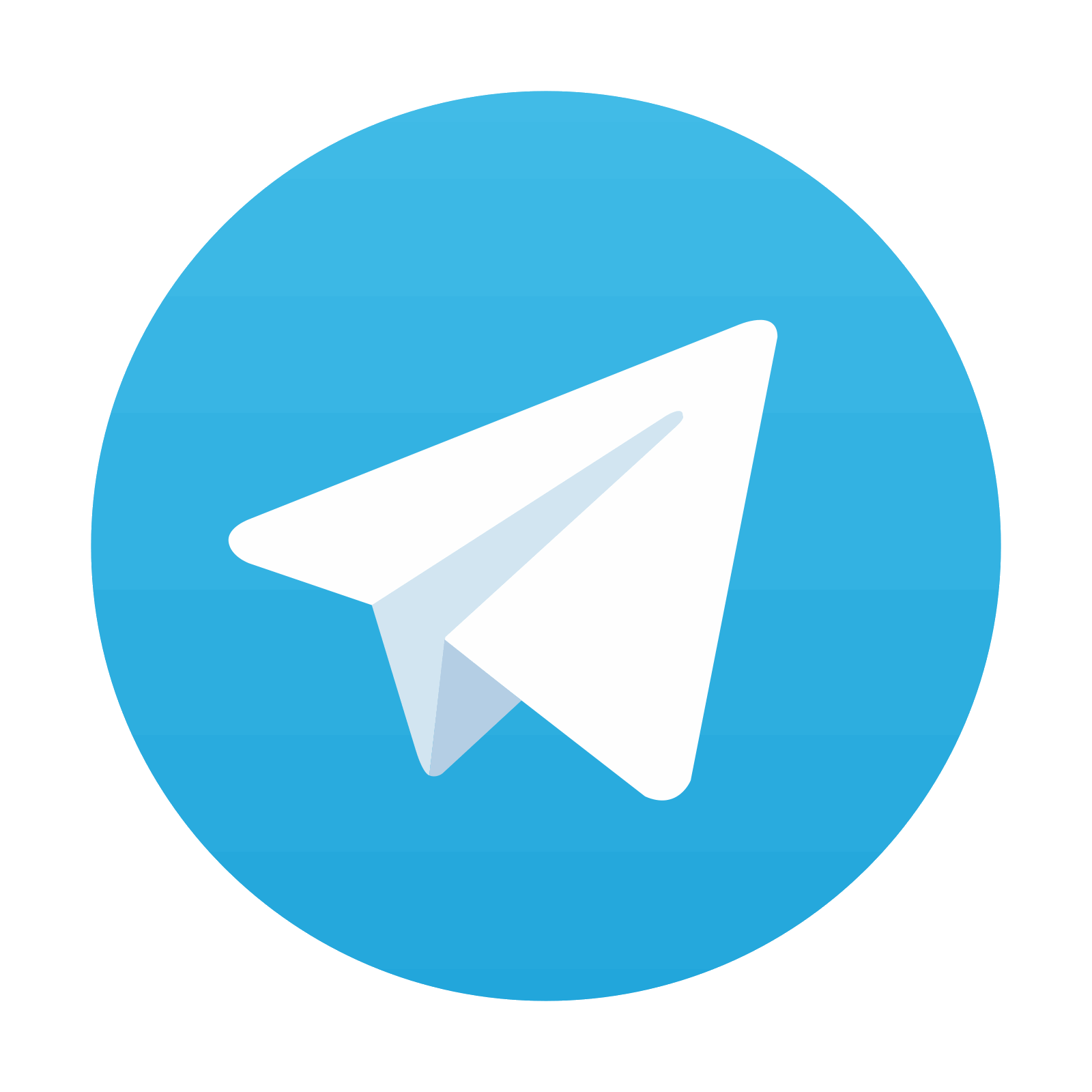
Stay updated, free articles. Join our Telegram channel
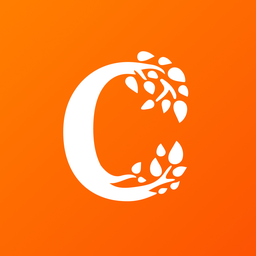
Full access? Get Clinical Tree
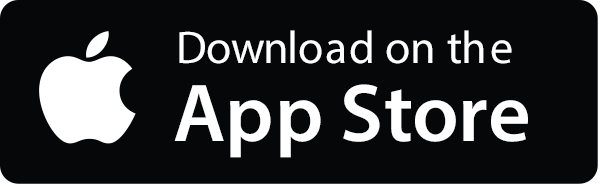
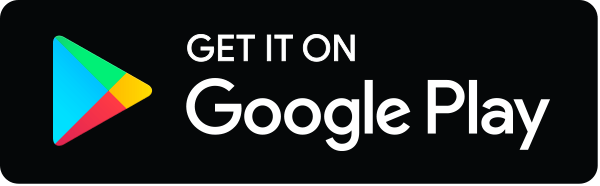