Chapter 45 Principles of Neurointensive Care
Clinical Assessment of Critically Ill Neurological Patients
The neurological examination for a NICU patient should always begin by defining the level and content of consciousness. Level of consciousness measures the degree of arousal or wakefulness of the patient. Scales are useful to facilitate communication and monitor changes over serial examinations; the Glasgow Coma Scale (GCS) is the most widely used (see Chapter 5, Table 5.4). However, it loses accuracy in patients who are intubated or develop cerebral ptosis (inability or only partial ability to open the eyes [by contracting the frontalis muscle] because a brain lesion impairs control of eye-opening mechanisms) and fails to provide information on brainstem function and respiratory status. A new scale (the FOUR score) that addresses these shortcomings has been validated in various patient populations and merits consideration as an alternative (Wijdicks et al., 2005) (Fig. 45.1). For patients with localized structural brain diseases, the National Institutes of Health (NIH) Stroke Scale may be used to grade and track focal neurological deficits.
In patients with altered consciousness, the results of one of these scales should be complemented with documentation of additional neurological features. Detailed description of the location and movements of the eyes, brainstem reflexes (pupillary light reactions, corneal, oculocephalic, oculovestibular, gag, cough), spontaneous movements and motor responses to pain, lateralizing signs, and breathing pattern must be recorded. In patients with delirium, the clinician must note the predominant behavioral abnormalities, degree of motor activity, and ability to interact with the environment. It is always important to dedicate special attention to any abnormal or adventitious movements, since seizures in critically ill patients may present with very subtle motor manifestations (e.g., nystagmoid eye movements). Fundoscopy may also offer valuable information and should be attempted; however, to avoid confounding future pupillary evaluations, mydriatic agents should not be administered. The reader is referred to Chapters 4 and 5 for further information relative to clinical evaluation of comatose and delirious patients.
Another essential aspect of the examination in critically ill patients is evaluating neuromuscular respiratory weakness. Timely recognition of signs of impending neuromuscular respiratory failure may avoid potentially devastating complications. Among them, use of accessory muscles and paradoxical breathing pattern are most indicative of problems. Paradoxical breathing is defined as the loss of synchronicity in chest and abdominal movements during respiration (i.e., abnormal sinking of the abdomen during inspiration) and represents an unequivocal sign of diaphragmatic failure (Rabinstein and Wijdicks, 2003b).
Monitoring in the Neurointensive Care Unit
Systemic Monitoring
Systemic monitoring in the NICU typically includes cardiac telemetry, frequent scheduled noninvasive BP measurements (by automatic cuff inflation) or continuous invasive arterial BP recording, pulse oximetry, and core body temperature. Continuous arterial BP monitoring is accomplished by inserting an indwelling cannula into a medium-caliber artery (e.g., radial arterial line). The invasiveness of the procedure is justified by the precise real-time information it provides. Continuous arterial BP monitoring is especially recommended in patients treated with induced hypertension (e.g., symptomatic vasospasm after SAH), cases requiring very strict BP control to avoid hemorrhagic complications (e.g., ruptured arteriovenous malformations), and patients with hypotension (e.g., sepsis), compromised cerebral perfusion pressure (CPP) (e.g., TBI with raised intracranial pressure [ICP]), or autonomic instability (e.g., Guillain-Barré syndrome). Arterial lines provide the additional advantage of eliminating the need for repeated arterial punctures to measure arterial blood gases. However, although generally safe, placement of an arterial line may be complicated by local infection, leading to bacteremia and thrombosis with risk of digital ischemia. Careful attention to proper technique and adherence to strict sterile conditions during placement and manipulation of the catheter are mandatory (Tegtmeyer et al., 2006).
The most accurate method of measuring core body temperature is a pulmonary artery catheter thermistor, but since most patients in the NICU do not require pulmonary artery catheter insertion, bladder or rectal probes are most frequently used. Bladder and rectal probes correlate well with pulmonary artery catheter thermistor readings, but there is a lag in the detection of temperature changes by the probes. The site of temperature recording becomes particularly important in patients treated with cooling measures. Thus, monitoring esophageal temperatures is recommended when using certain intravascular cooling devices (De Georgia et al., 2004).
Central venous catheters allow monitoring of central venous pressure while providing access for fluid and drug administration. They are, however, a frequent source of infection. Rigorous sterile techniques at the time of catheter insertion, cutaneous antisepsis with chlorhexidine (rather than povidone-iodine), topical application of antiinfective ointment or a chlorhexidine-impregnated dressing to the insertion site, and catheters with an antiinfective surface may reduce the risk of catheter-related bloodstream infection (Safdar et al., 2002). The role of pulmonary artery catheters in ICUs is shrinking as studies consistently demonstrate that their use is associated with higher rates of complications (Sandham et al., 2003; Wheeler et al., 2006).
Brain Monitoring
Multiple brain monitoring methods are now available. They are most useful when applied in combination, a practice known as multimodality monitoring (Diedler and Czosnyka, 2010). It is important to be aware, however, that the endpoints of most studies validating the use of brain monitoring methods modalities have been surrogate physiological measures rather than actual assessments of patients’ functional outcome. In fact, there is no class I evidence proving that the use of multimodality brain monitoring results in improved clinical outcomes. Currently, the clinical application of brain monitoring techniques is restricted to large centers, especially those treating numerous TBI patients.
Methods for cerebral monitoring are divided into three main categories according to their spatial resolution: global, regional, and local brain monitoring (Table 45.1). Global brain monitoring techniques measure ICP, CPP, electrical potentials, and venous oxygen saturation. Regional and local brain monitoring methods include cerebral blood flow (CBF), cerebral blood flow velocities (BFV), brain tissue metabolism, temperature, and oxygenation.
Global Brain Monitoring Techniques
Intracranial Pressure Monitoring
The intracranial space is occupied by three constituent compartments: the brain (accounting for 80% to 90% of the intracranial volume), the blood, and the cerebrospinal fluid (CSF). Because the skull is rigid, any expansion of one of these compartments must be compensated by a reduction in size of the others (a physiological principle known as the Monro-Kellie doctrine). When this compensation is insufficient, the ICP rises. Small increases in intracranial volume can be initially accommodated with little or no effect on the ICP, but as more volume is added, intracranial compliance falls until it reaches a critical point beyond which a minimal increase in volume causes an exponential rise in ICP. This pressure-volume relationship is depicted in Fig. 45.2. In other words, the initial physiological response to an increase in brain volume is a reduction in the CSF and venous blood volumes by shifting these fluids out of the intracranial space (except in the cases of hydrocephalus and venous thrombosis). Once these compensatory mechanisms are exhausted, the system becomes noncompliant, and further increments in brain volume compromise arterial blood flow and eventually lead to herniation of brain tissue.
It has also been argued that the main purpose of ICP monitoring is maintenance of normal CPP (normal, >70 mm Hg) because the latter may be more related to secondary ischemic injury (Rosner et al., 1995). The relative importance of ICP and CPP as main targets of therapy remains a matter of debate.
ICP is pulsatile and has systolic and diastolic components. In addition to the value of mean ICP, these components must also be evaluated carefully. The normal ICP waveform consists of a 3-peaked wave (Fig. 45.3). P1, the first and generally the tallest peak, is also known as the percussion wave and corresponds to the transmitted systolic BP; P2 (the tidal wave) and P3 (the dicrotic wave) are normally smaller peaks, and the notch between them corresponds to the dicrotic notch of the arterial waveform. As ICP increases, P2 and P3 rise and eventually surpass P1. Ultimately, with continued elevation of ICP, the waveform loses distinct peaks and assumes a triangular morphology. Intracranial pathology leading to sustained elevations of ICP may produce plateau waves, also known as A-waves of Lundberg (see Fig. 45.3). These waves reflect a sudden dramatic rise in ICP to levels of 40 to 100 mm Hg, often lasting 5 to 20 minutes. Plateau waves indicate critically low intracranial compliance leading to marked changes in ICP, even with very small variations in intracranial volume. Although their pathophysiology is not fully elucidated, experimental observations suggest that plateau waves may be generated by brief episodes of systemic hypotension leading to exaggerated cerebral vasodilation in patients with abnormal vasomotor reactivity (Rosner and Becker, 1984).
ICP may be monitored using intraparenchymal, intraventricular, epidural, or subdural devices (Brain Trauma Foundation, 2007). Intraventricular monitoring remains the gold standard because of its precision. It consists of a ventricular catheter connected to an external transducer which allows continuous ICP readings. Advantages of this technique are providing reliable ICP measurements and allowing external drainage of CSF. Hence, ventricular monitoring is indicated in patients with hydrocephalus and often preferred in those with refractory intracranial hypertension. Major drawbacks are higher risk of infection (rate of ventriculitis is 3% to 8% and increases with duration of the ventriculostomy) (Flibotte et al., 2004; Holloway et al., 1996; Martinez-Manas et al., 2000), risk of bleeding at the time of catheter placement (especially in patients with underlying coagulopathy or recent use of antithrombotics), and system malfunction (dampening of the waveform may be caused by apposition of the catheter tip against the ventricular wall or obstruction of the catheter by a blood clot or an air bubble). Risks may be minimized by careful placement of the catheter and maintenance of the system under strict sterile conditions, use of antibiotic prophylaxis (e.g., cefotaxime 2 g every 6 hours from the time of catheter insertion until 24 to 48 hours after its removal) (Flibotte et al., 2004), and withdrawal of the catheter as soon as possible (Holloway et al., 1996). Exchange of the catheter every 5 days, although a common practice, does not appear to decrease the risk of infection (Holloway et al., 1996; Lozier et al., 2002); in fact, repeated catheter insertions have been found to be associated with higher risk of ventriculitis (Arabi et al., 2005).
Intraparenchymal fiberoptic monitors are also quite accurate. When compared with intraventricular catheters, the measurements provided by intraparenchymal monitors differ on average by ± 2 to 5 mm Hg. Advantages of this monitoring system include simple and safe insertion technique, easy maintenance, relative lack of substantial drift (even after several days), and low risk of infection. Disadvantages include high cost, technical complications (e.g., breakage of the optical fiber), and most importantly, inability to drain CSF. Epidural and subdural monitors are less reliable and therefore rarely used but are a valuable option for patients with severe coagulopathy (e.g., liver failure with cerebral edema), given their lower risk of hemorrhagic complications (Vaquero et al., 2005).
ICP should be monitored in patients with severe TBI and a GCS sum score below 9 and an abnormal computed tomography (CT) scan, or a normal CT scan with two or more of the following criteria: age older than 40, unilateral or bilateral motor posturing, and systolic BP less than 90 mm Hg (Brain Trauma Foundation, 2007). It is difficult to extrapolate the value of these guidelines to patients with diagnoses other than trauma, owing to lack of specific data on ICP monitoring in those other conditions. Some experts advocate monitoring ICP in comatose patients with a large intracranial mass lesion (hematoma, abscess, large infarctions, etc.) causing radiologically documented tissue shift. Patients with SAH, ICH, or cerebellar ischemic or hemorrhagic strokes producing acute hydrocephalus typically have their ICP monitored once a ventriculostomy catheter has been placed primarily for drainage purposes.
Jugular Bulb Oximetry
Jugular bulb oximetry measures the oxygen saturation of venous blood returning from the brain (normal 50% to 65%) by means of a fiberoptic catheter (Feldman and Robertson, 1997). The main goal of jugular venous oxygen saturation (Sjvo2) monitoring is to provide a continuous measure of the changing balance between cerebral oxygen delivery and cerebral oxygen consumption. Simultaneous determination of Sjvo2 using the jugular bulb catheter and arterial oxygen saturation (Sao2) allows for the calculation of the intracranial arteriovenous oxygen difference (AVDo2) (normal 24%-42%). Cerebral oxygen consumption can be calculated as the product of AVDo2 and CBF. The cerebral oxygen extraction rate (O2ER) is derived from the ratio of cerebral oxygen consumption to cerebral oxygen delivery.
Jugular venous desaturations denote relative reductions of global cerebral oxygenation. Sjvo2 below 50% for 15 minutes or more are deemed indicative of ischemia. Sjvo2 monitoring has been mostly tested in patients with severe TBI. In these patients, jugular venous desaturations have been shown to correlate with the occurrence of secondary brain insults and poor outcome (Gopinath et al., 1994; Robertson et al., 1995). High Sjvo2 should not simply be equated with hyperemia; it may also be associated with poor outcome in comatose patients, possibly indicating lack of oxygen utilization after extensive neuronal death (Cormio et al., 1999). Favorable experience with jugular bulb oximetry has been reported in patients with SAH and ICH (Heran et al., 2004; von Helden et al., 1993), but interpreting Sjvo2 may be difficult in patients with severe hemispheric ischemic strokes (Keller et al., 2002). This technique is also used to monitor cerebral oxygenation during neurosurgical procedures.
Therapeutic interventions in response to information provided by jugular bulb oximetry have been proposed, including adjustment of the degree of hyperventilation, timing and intensity of osmotherapy, adjustment of MAP, and treatment of anemia (Macmillan and Andrews, 2000). There is no proof, however, that these interventions improve functional outcome. As shown by the negative results observed in studies testing therapies guided by pulmonary artery catheters, the clinical value of aggressive interventions aimed at optimizing physiological parameters must be proven before we incorporate these into clinical practice.
Advantages of the jugular bulb catheter as a monitoring modality include the practicality of continuous bedside monitoring, the capability of confirming the oximeter reading by drawing blood through the catheter, and the numerous physiological parameters that can be derived from the Sjvo2 to ascertain cerebral oxygen balance. Disadvantages of the catheter include its susceptibility to positioning artifacts and the complications associated with catheter insertion, including carotid puncture, infection, accidental misplacement, and jugular thrombosis (Coplin et al., 1997; Coplin et al., 1998; Latronico et al., 2000).
Electroencephalography
Continuous bedside electroencephalography (EEG) monitoring is based on four of its major neurobiological features (Jordan, 1995): (1) its close relationship to cerebral metabolic rate; (2) its sensitivity in detecting hypoxic-ischemic neuronal dysfunction at an early stage; (3) its obvious primacy as a monitor of seizure activity; and (4) its value in cerebral localization. Continuous EEG recording has been advocated as a valuable routine tool to monitor critically ill neurosurgical and neurological patients.
Status epilepticus is the most common indication for EEG monitoring, because the clinical ascertainment of ongoing seizure activity is often obscured by the effect of sedatives and analgesic agents. The EEG is essential for monitoring the effects of treatment, especially when barbiturates or general anesthetics are administered to achieve a burst-suppression pattern. Detection of nonconvulsive seizures and nonconvulsive status epilepticus (NCSE) can only be accomplished by EEG monitoring. Timely diagnosis of NCSE is important because delayed recognition may be associated with increased mortality (Young et al., 1996).
Nonconvulsive seizures were reported in up to one-third of unselected NICU patients, frequently involving the presence of NCSE (Jordan, 1995). NCSE has also been noted in 8% of comatose patients in medical ICUs (Towne et al., 2000), and nearly a third of septic patients with encephalopathy may have electrographic seizures without obvious clinical manifestations (Oddo et al., 2009). Continuous EEG monitoring has documented nonconvulsive seizures after severe TBI, ischemic stroke, poor-grade SAH, ICH, and after termination of generalized convulsive status epilepticus (DeLorenzo et al., 1998; Dennis et al., 2002; Vespa et al., 1999). These events might exacerbate excitotoxic injury in vulnerable brains and have been associated with high mortality (Young et al., 1996). But while their prognostic value is fairly well established, the impact of aggressive treatment of nonconvulsive seizures on clinical outcome remains to be determined (Hirsch, 2004).
Continuous EEG monitoring has also been used as an aid for early detection of ischemia in patients with SAH at high risk for vasospasm. Although early experience is promising (Claassen et al., 2006; Vespa et al., 1997), it is too early to recommend continuous EEG for this indication. Intracortical EEG (based on the use of deep electrodes) may be substantially superior to scalp EEG for detecting changes related to secondary neurological insults in patients with various forms of acute brain injury (Waziri et al., 2009). Furthermore, recurrent cortical spreading depolarizations may exacerbate local brain hypoxia in patients with TBI or SAH (Bosche et al., 2010), but the value of monitoring for these changes with intracortical EEG remains to be conclusively determined.
Other applications of EEG in the ICU, especially in comatose patients, include evaluating metabolic encephalopathy (EEG serves to substantiate the diagnosis by showing diffusely slow, low-amplitude activity, and often triphasic waves, but does not distinguish between different causes of the condition), recognizing psychogenic unresponsiveness, and confirming brain death (Wijdicks, 2001). After cardiac arrest, near-complete suppression, burst-suppression, nonreactive alpha or theta rhythms (alpha or theta coma), status epilepticus and generalized periodic complexes are considered malignant patterns (Rossetti et al., 2007; Synek, 1990). Although valuable for the prognostication of anoxic-ischemic encephalopathy, EEG data should not be interpreted in isolation in these patients (Wijdicks et al., 2006).
Evoked Potentials
Evoked potentials have a more restricted role in the NICU (Moulton et al., 1998). The median nerve somatosensory evoked potential (SSEP) has been mostly used; technical details are discussed in Chapter 32A. Bilateral absence of the N20 response 1 to 3 days after cardiopulmonary resuscitation accurately predicts poor chances of recovery of awareness (Zandbergen et al., 2006). Unfortunately, presence of these responses after anoxic brain injury lacks meaningful prognostic value.
Regional/Focal Brain Monitoring Techniques
Transcranial Doppler Ultrasonography
TCD ultrasonography is a noninvasive technique used to evaluate mean CBF velocity in the large intracranial arteries at the level of the circle of Willis. TCD is easy to learn and use, noninvasive, and safe. It measures CBF velocity rather than CBF, and the linear relationship between CBF and BFV depends on the angle of insonation. Still, TCD provides a wealth of useful clinical information including the presence or absence of blood flow, its velocity (systolic, diastolic, and mean), and direction. It also allows calculation of the pulsatility index (PI = peak systolic velocity minus end diastolic velocity divided by mean BFV), which represents the downstream resistance to blood flow. Increases in BFV are observed in patients with cerebral vasospasm, hyperventilation (which produces vasoconstriction), and anemia. Cerebral vasospasm may be distinguished from hyperdynamic status by measuring the hemispheric index or Lindegaard ratio (ratio of middle cerebral artery to extracranial internal carotid artery mean BFV) (Lindegaard et al., 1989). A ratio greater than 3 is considered indicative of vasospasm; a low ratio is more suggestive of hyperemia. TCD also allows assessment of vasomotor reactivity (Ng et al., 2000). Impairment of vasomotor reactivity is a well-established poor prognostic factor in patients with TBI and may portend the occurrence of symptomatic vasospasm in patients with SAH (Czosnyka et al., 1997; Frontera et al., 2006b). TCD may also be used as a confirmatory test for the diagnosis of brain death (severely diminished mean cerebral BFV associated with absent diastolic flow, reversed flow, and severely elevated PI).
The diagnosis of cerebral vasospasm in patients with SAH remains the main indication of TCD monitoring in the NICU. The criteria for vasospasm in the middle cerebral artery territory is a mean BFV greater than 120 cm/sec with a hemispheric index greater than 3, or an increment greater than 50 cm/sec within a 24-hour period (Suarez et al., 2002). A specialized headset allows continuous monitoring of BFV and may be a useful adjunct in monitoring patients at high risk for vasospasm. TCD monitoring in patients with cerebral vasospasm has good correlation with angiographic vasospasm and is comparable to conventional angiography in the prognostication of delayed ischemia in these patient, although neither technique is uniformly diagnostic (Rabinstein et al., 2004).
Local Cerebral Oxygenation Monitoring Techniques
Brain tissue oxygen probes and near-infrared spectroscopy allow assessment of local oxygenation. Brain tissue oxygen may be measured by invasive probes such as the Licox catheter. Apart from tissue Po2, this catheter allows measurement of brain temperature. Brain tissue Po2 measures the diffusion of dissolved plasma oxygen across the blood brain barrier (rather than CBF, arterial delivery of oxygen, or brain metabolism) in a relatively small area of brain tissue (approximately 15 mm3) (Rosenthal et al., 2009). Factors that determine brain tissue Po2 include Pao2, arterial Paco2, systemic BP, and CBF. Normal brain tissue Po2 values range from 25 to 30 mm Hg. The major disadvantages of brain oxygen probes include their invasiveness, limited spatial resolution, and susceptibility to artifacts (due to inappropriate calibration and head movement, among other factors). Its use has been recommended by experts in various major centers for patients with severe head injuries and poor-grade SAH (Maloney-Wilensky et al., 2009). It is best used when applied in the setting of multimodality monitoring, along with jugular oximetry and perhaps microdialysis (Andrews et al., 2008) (Fig. 45.4).
Near-infrared spectroscopy (NIRS) is based on the property of a near-infrared light (700–1000 nm) to pass through tissues while being both scattered and absorbed. The absorption of a near-infrared light is proportional to the local concentration of certain chromophores, most notably hemoglobin. Thus, the absorption of near-infrared light changes according to the oxygenation state of hemoglobin. The probes illuminate up to a volume of 10 mL of brain tissue. All measurements are expressed as absolute concentration changes from a baseline zero at the start of the measurement. Normal values of oxygenated hemoglobin are reported to be 60% to 80%, and ischemic threshold is estimated to be below 47% saturation (Casati et al., 2006). However, the reliability of this technique has been questioned. It is susceptible to extraneous light, motion artifact, and signal drift. The measurement may also become unreliable when obtained through intracranial hematomas or through blood in the CSF.
Microdialysis
The basic concept of microdialysis involves inserting a fine catheter into the brain parenchyma, then perfusing the catheter with a physiological solution such as Ringer’s lactate, thereby facilitating the exchange of molecules between the perfusate and the extracellular fluid across a dialysis membrane located within the catheter tip. The dialysate is sampled under sterile conditions at hourly or other regular intervals and put through a microdialysis analyzer at the bedside. Insertion artifacts make measurements unreliable for the first hour after placement (Bellander et al., 2004).
Microdialysis allows monitoring of brain pH, lactate and pyruvate, glucose, glycerol, glutamate, urea, and potentially other soluble molecules of interest (Bellander et al., 2004; Nilsson et al., 1999; Vespa et al., 1998). Changes in lactate concentration, lactate/pyruvate ratio, and glutamate concentration have been used as indices of cerebral ischemia. A lactate/pyruvate ratio greater than 25 is probably the best indicator of ischemia (Andrews et al., 2008; Bellander et al., 2004). Rises in glycerol are believed to reflect phospholipid breakdown as a result of cell membrane damage. Because of this, cerebral microdialysis has been employed in the NICU to monitor for cerebral vasospasm and delayed cerebral ischemia in SAH, to identify secondary insults after severe brain trauma, and to follow extracellular glutamate concentration peri-ictally in patients with epilepsy.
Several aspects of microdialytic analysis remain controversial, such as where to place the catheter (Andrews et al., 2008), whether the lactate/pyruvate ratio alone or in combination with other parameters is a better indicator of early cerebral ischemia, and why there has been no correlation between microdialysis measures and clinical outcome in some studies. Other problems are the invasiveness and labor intensiveness of the technique. It currently represents a valuable research tool, but its widespread clinical use cannot yet be recommended.
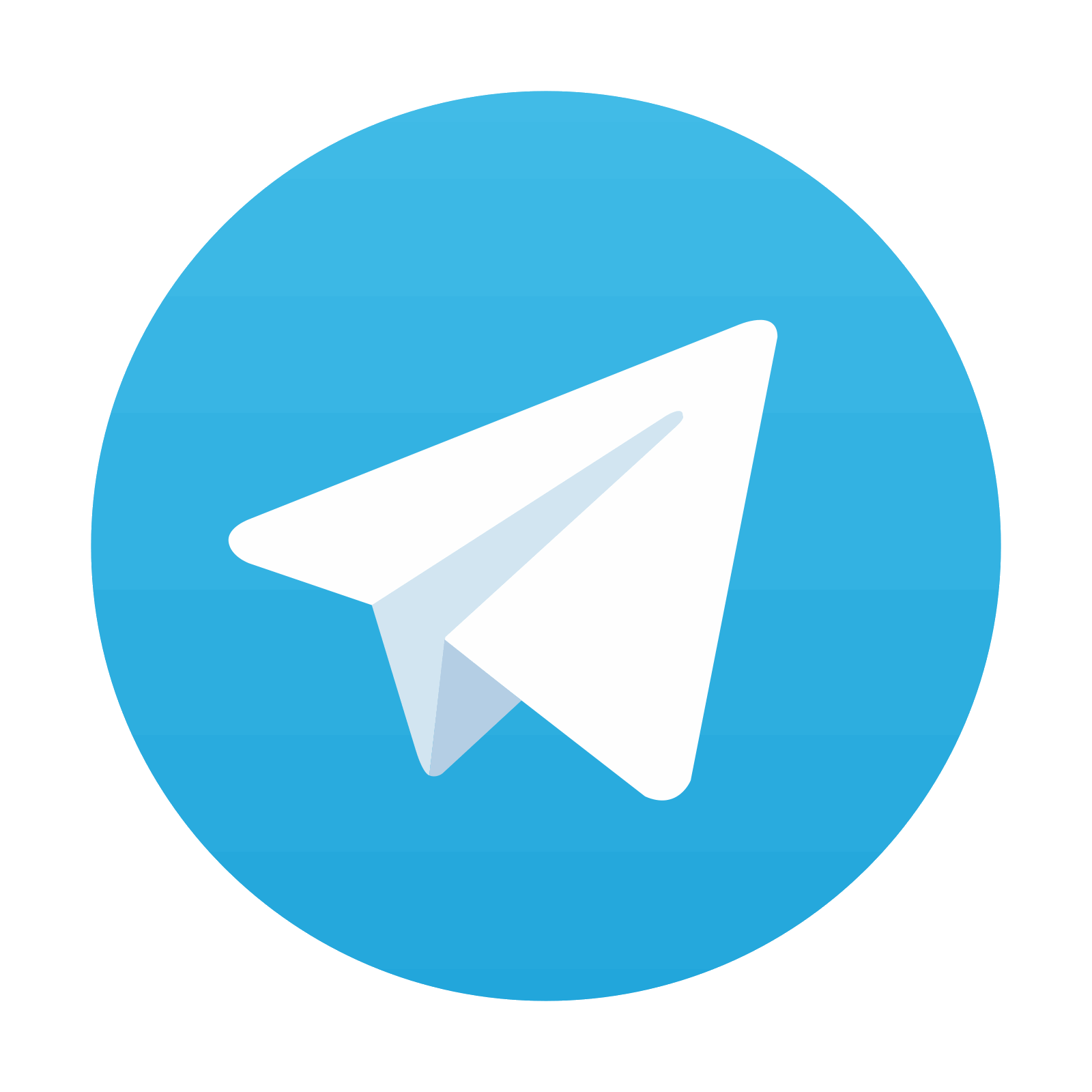
Stay updated, free articles. Join our Telegram channel
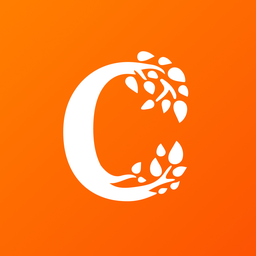
Full access? Get Clinical Tree
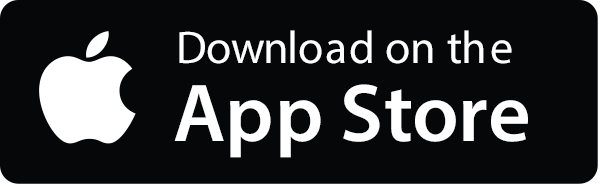
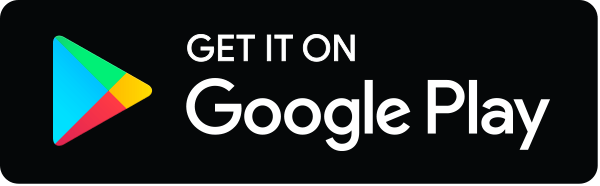