Chapter 7 Respiratory function testing is common practice in the medical setting as it helps to identify disease and disability and may clinically indicate why a patient is complaining of shortness of breath or having difficulty ventilating. Typically, a combination of pulmonary function tests is used to help differentially diagnose a patient’s respiratory problem. And although appropriate referral to a pulmonologist is necessary to diagnose a pulmonary disease, there are general principles every clinician should be familiar with regarding pulmonary function, pulmonary function screening procedures, and interpretation of pulmonary function outcome variables. Individual pulmonary function tests give information regarding specific lung functions. Disruptions of lung function can look similar across distinct pathologic conditions. Subsequently, there are times when more detailed pulmonary function tests must be pursued to manage the patient effectively. This chapter provides an overview of ventilation, and reviews the pulmonary function tests and screening procedures. The primary biologic purpose of ventilation is gas exchange between the body and the atmosphere. Oxygen (O2) is transported from the atmosphere into the lungs and ultimately to body tissues. Carbon dioxide (CO2) needs to be carried from the tissues, to the lungs, and expelled from the body. Gas exchange begins with inspiration. Air flows into the mouth, through the upper airway, larynx, and into the trachea. The trachea divides into two main stem bronchi at the carina, which in turn continue dividing into smaller bronchi, bronchioles, and the terminal bronchioles. The terminal bronchioles then divide into respiratory bronchioles, alveolar ducts, and alveolar sacs.1–5 The trachea, bronchi, and bronchioles comprise the conducting airways, and are sometimes termed “anatomic dead space,” because no gas exchange occurs in those structures. The primary role of the conducting airways is to warm, clean, and humidify atmospheric air before it reaches the alveoli. Respiratory bronchioles, alveolar ducts, and alveolar sacs comprise the alveolar air space, and this is where gas exchange of O2 and CO2 takes place.2,3,6 Because gas exchange occurs within the alveolar air space, the volume of air that moves into the alveoli (alveolar ventilation; VA) from the atmosphere is very important. On average, approximately 500 mL of air enters the airway with each breath (tidal volume; TV). Of this, 150 mL remains in the conducting airways and 350 mL reach the alveoli. Tidal volume multiplied by the number of breaths per minute (on average, 12) is equal to the minute ventilation (mL/min). Minute volume ventilation refers to the amount of air that is moved in and out of the lungs in a minute2,5,7,8 and can be altered by either changing the number of breaths per minute or by changing the TV. Either one of these parameters is relatively easy to manipulate, and both are important, for example, when considering ventilator settings for ventilator-dependent patients. If ventilator settings are such that there is asynchrony between respiratory muscle contraction and the respiratory cycle timing imposed by the ventilator, gas trapping, or failure to move O2 and CO2 in and out of the lungs, may occur.9–12 To calculate alveolar ventilation (VA), or the amount of air reaching the alveoli, simply subtract the volume of air in the conducting airways (150 mL) from the TV (500 mL), and then multiply by the number of breaths per minute. Thus, for an average healthy adult male breathing at a rate of 12 breaths per minute, the VA is 4200 mL/minute [12 breaths/min × (500 mL – 150 mL)]. That is, 4200 mL of air reaches the alveoli per minute of tidal breathing. This is an important value when considering a person’s ability to adequately oxygenate the body tissues. Decreased alveolar ventilation may result from several pathologies, including airway obstruction (i.e., asthma, chronic obstructive pulmonary disease [COPD], laryngeal cancer) or decreased inspiratory drive (weakness in the muscles of inspiration). Alveolar ventilation can be increased by either increasing the respiratory rate (minute ventilation) or by increasing TV. Because increasing respiratory rate can lead to hypoxemia (discussed later), TV is typically thought of as the more important factor in determining how well the alveoli are ventilated.3,5–7 Atmospheric air is composed of a mixture of gases, including O2, CO2, nitrogen (N2), and trace amounts of water vapor. Dalton’s law of gases indicates that the total pressure exerted by a mixture of gases is equal to the total of partial pressures exerted by each gas in the mixture.2,5,6,8 The partial pressure of an individual gas is dependent on the percentage concentration of that gas in the mixture. At sea level, atmospheric pressure, or barometric pressure (PB) of atmospheric air, is 760 mm Hg. Oxygen makes up approximately 20.8% of atmospheric air, and therefore the partial pressure of oxygen (Po2) at sea level is 158 mm Hg (20.8% × 760 mm Hg). See Table 7.1 for partial pressures of gases comprising atmospheric air.2,7
Pulmonary Function Evaluation
Ventilation
Overview
Changes of Partial Pressures of Atmospheric Gases During Ventilation
Percentage Concentration | Partial Pressure (mm Hg) | |
Nitrogen (N2) | 79% | 600.40 |
Oxygen (O2) | 20.8% | 158.08 |
Carbon dioxide (CO2) | Trace | 0.30 |
Water (H2O) | Trace | 0.00 |
Total atmospheric | 760.00 |
As air flows from the atmosphere to the alveoli, the composition of the gas mixture changes. Inspired air is warmed and humidified; as this occurs approximately 47 mm Hg of water vapor is added to the mixture. Thus, the adjusted PO2 of inspired air (PIo2) is 150 mm Hg [(760 − 47) ×20.8%]. Subsequent mixing of inspired air with air already in the lungs also changes the partial pressures. An estimate of the partial pressure of oxygen in the alveoli (PAo2) is given by the alveolar gas equation:
PA02 = PI02 − (PAco2/R), where R is a constant equal to 0.8.
Thus, the PAO2 at sea level is 100 mm Hg [150 −(40/0.8)]. It is the PAO2 that is important in examining the oxygenating capacity of the lungs.2,5,7 PAO2 is an indirect measure of arterial Po2 (Pao2), which gives information about gas exchange between the alveoli and alveolar capillaries. A decreased PAo2 will lead to a decreased Pao2, which leads to decreased oxygen delivery to the tissues. In the case of normal PAO2 (100 mm Hg) and decreased PaO2, the problem likely exists at the level of the actual gas exchange across the alveolar and capillary membranes, as is the case in intrinsic pulmonary diseases, such as emphysema.8,13
The partial pressures change in the blood after gas exchange takes place across the alveolar and capillary membranes, in the tissues, and in the venous return blood after cellular respiration has occurred. A summary of partial pressures of O2 and CO2 from atmosphere to lungs, blood, tissue, and back to the lungs is given in Fig. 7.1 . Keep in mind that as the PB changes, so does the partial pressure of each gas.5,7,13–16 For example, at an altitude of 10,000 feet PB is 523 mm Hg. The percentage composition of oxygen remains the same, and the PO2 is 110 mm Hg (20.8% ×523 mm Hg) as compared with 158 mm Hg at sea level. Pulmonologists, respiratory physiologists, and clinicians involved in respiratory monitoring must attend to this important information. Normal values undergo a change at altitude, which must be considered when planning therapeutic intervention. Partial pressures in the alveoli and arterial blood are lower at higher altitudes. This is problematic for some patients, especially those with already compromised respiratory function. In these cases, giving oxygen can increase the partial pressures and subsequently improve the oxygenating capacity of the lungs and oxygen delivery to the tissues.17
Fig. 7.1 Partial pressures in the alveoli, in the blood entering and leaving the alveolar capillaries, and in the body tissues (sea level).
Gas Exchange
Once inspired air has reached the alveolus, the airflow has slowed and the predominant means of air movement is diffusion. The rate of diffusion is governed by Fick’s law of diffusion. Fick’s law of diffusion states that the rate of diffusion of a gas across a membrane is directly proportional to the differences in partial pressure (partial pressure gradient), and to the area of the membrane, and inversely proportional to the thickness of the membrane. In more general terms, the rate of diffusion increases with increases in partial pressure gradients and increasing area of the membrane. The rate of diffusion decreases with increasing membrane thickness. Fick’s law is given by the following equation:
where J is the rate of molecular movement, D is the permeability constant of the membrane, A is the area of the membrane, α is the solubility of the gas, P is the concentration gradient (partial pressure gradient), and X is the thickness of the membrane.4,5,18–20 In terms of respiratory physiology, the rate of diffusion, J, for gas consumption is the consumption rate of oxygen (VO2) or the release rate of CO2, (VCO2).
The blood entering the capillaries from the tissues, or venous return blood, contains O2 and CO2 with partial pressures of 40 mm Hg and 46 mm Hg, respectively. In the alveoli, the PAO2 is 100 mm Hg, and the PACO2 is 40. Thus, partial pressure gradients are set up between alveolar and venous oxygen and CO2, resulting in a net diffusion of oxygen into the blood and CO2 into the lungs. The membrane separating the alveolus and the blood is very thin, approximately 0.5 mm. In the lung, A, D, and X are typically constant, and they can be combined into a term D L, which is called the diffusing capacity. Thus, the rate of diffusion for respiratory gases can be expressed as:
Because many diseases result in diffusion impairment by their effects on membrane thickness (pulmonary edema) or surface area (such as emphysema), determining the D L is a useful tool to diagnose diffusion impairment that can result in low arterial oxygen and high arterial CO2.4,5,18–20 For example, with pulmonary edema, Fick’s law indicates that the rate of diffusion is decreased secondary to increased membrane thickness, resulting in decreased oxygen delivery to the tissues and decreased CO2 extracted from venous return blood.
Once this gas exchange between the alveoli and pulmonary capillaries occurs, the freshly oxygenated blood is routed back through the left heart and subsequently pumped to body tissues where cellular respiration takes place. As a result of cellular respiration, O2 is consumed by the tissue in the production of adenosine triphosphate (ATP), and CO2 is created as a waste product. Carbon dioxide then plays a role in blood buffering and keeping an appropriate acid–base balance in the body.2,3,5,7,21,22
Ventilation/Perfusion Matching
Pulmonary circulation sends the total cardiac output through the pulmonary vasculature, where the gas exchange takes place. Blood accumulates in the right heart after returning from systemic circulation, where it has accumulated CO2 and released O2 in the distal tissues. The right heart provides the energy, or pump, to drive blood flow into pulmonary capillaries. Blood flows through the pulmonary capillary typically at a rate that results in a 0.75-second transit time past the alveoli. Fortunately, blood reaches an equilibrium with the alveolar gas levels (exchange of O2 and CO2) in approximately 0.25 seconds, so there is a built-in safety buffer in the transit time that helps ensure equilibrium will occur even if the flow rate should change.2,7,8,23–25
Pulmonary blood flow is measured by perfusion, denoted “Q.” That is, Q is a measure of how much blood is flowing to the lungs. The ratio of ventilation to perfusion is called the V A/Q ratio. The V A/Q ratio provides an estimate of the match between blood perfusion of a pulmonary capillary around an alveolus and the ventilation of the alveolus. In healthy individuals the V A/Q should be approximately 1.0, indicating a match between ventilation and perfusion. A high V A/Q is indicative of good ventilation but poor perfusion. Conversely, a low V A/Q indicates poor ventilation and good perfusion. High and low V A/Q both result in inadequate gas exchange.1,23,25–30 Many clinical symptoms, including shortness of breath, wheezing, coughing, nausea, and dizziness, can be manifestations of V A/Q mismatch. Respiratory, cardiac, or metabolic disorders are all suspects in a patient with these symptoms, and appropriate referrals should be made.21
Hypoxemia
Matching O2 and CO2 levels in the alveoli with levels in the blood is paramount for adequate removal of CO2 and oxygenation. This means there must be an appropriate match between ventilation and perfusion (e.g., V A/Q = 1.0). A mismatch results in hypoxemia, which is a decrease in PaO2.1,5,8,23,25,29 Hypoxemia can be considered in four main categories, which are discussed in the following subsections.
Hypoxic Hypoxemia
Hypoxic hypoxemia is due to a decrease in PIO2 and can result from decreased PB, as occurs at high altitudes. This results in decreased PO2 and subsequent decreased PIO2. Additionally, decreased PIO2 may occur at normal PB, but in cases where the gas has decreased oxygen content (i.e., <20.8%) due to dilution by other gases. In either scenario, PIO2 is reduced, leading to decreased PAO2 and low PaO2. This may be helped by increasing the PO2 of inspired air.5,19,21,25,27,30
Alveolar Hypoventilation
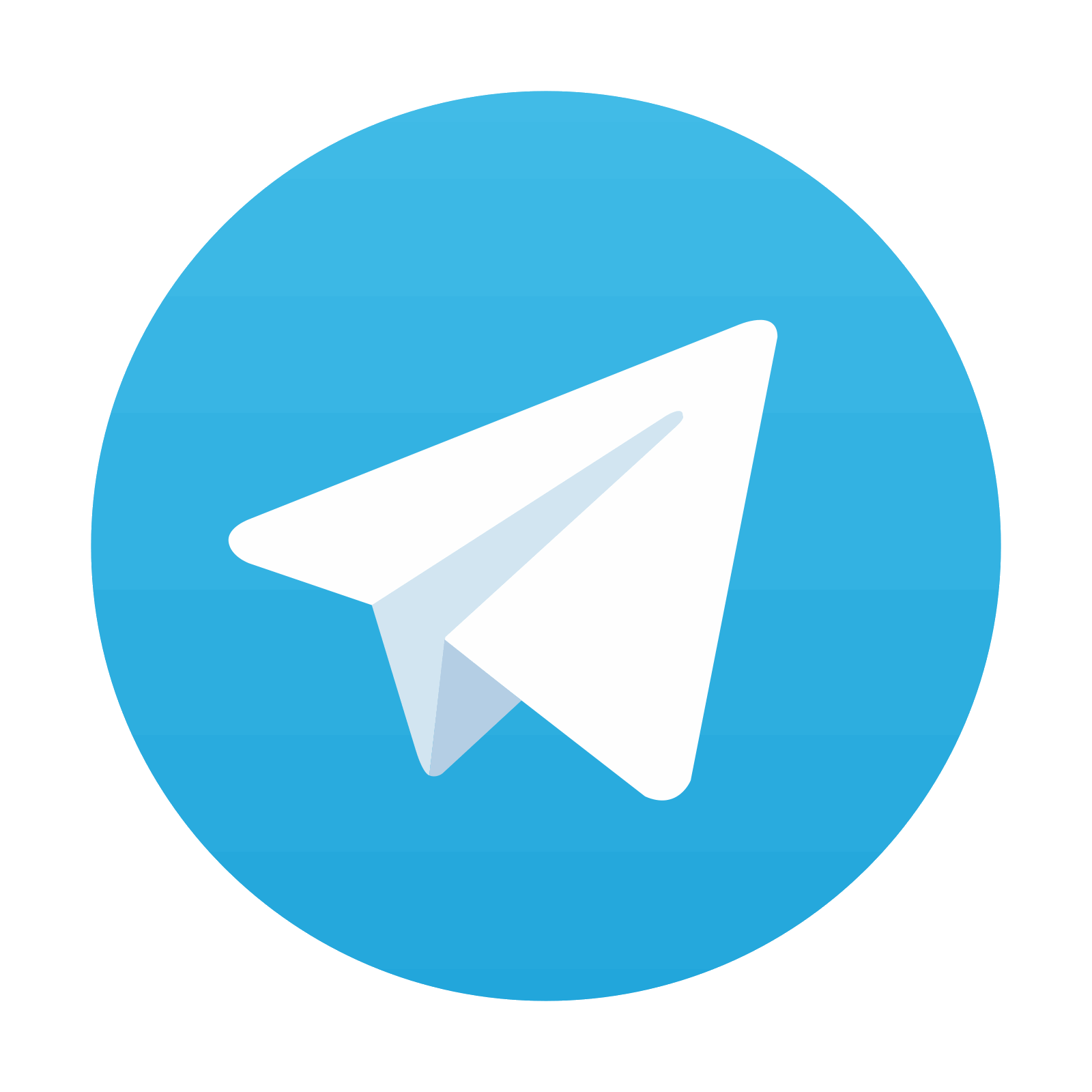
Stay updated, free articles. Join our Telegram channel
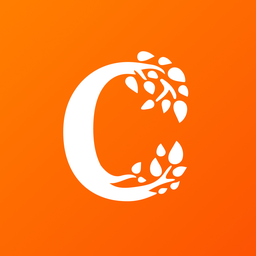
Full access? Get Clinical Tree
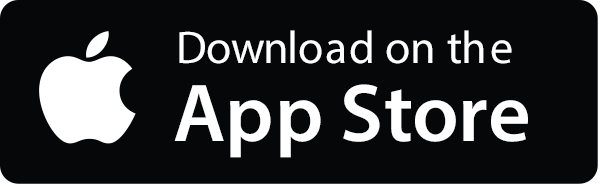
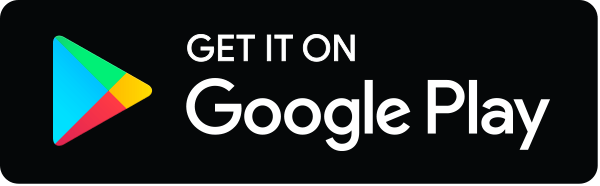