and Robert E. Schmidt2
(1)
Sunnybrook and St Michael’s Hospitals, University of Toronto, Toronto, ON, Canada
(2)
Division of Neuropathology Department of Pathology, Washington University School of Medicine, St. Louis, MO, USA
5.1 Normal Structure and Function of Schwann Cells and Myelin
Schwann cells (SCs) are derived from the neural crest (Webster 1993) and encircle all peripheral nerve axons. Functionally, two populations of Schwann cells can be identified, those producing myelin and those associated with unmyelinated axons. Because this distinction is fundamental to the appearance and physiology of SCs, the two types will be discussed separately. Schwann cells associated with unmyelinated fibers have been called Remak cells, but we will refer to them as nonmyelinating Schwann cells (NMSCs) because whether an SC produces myelin or not depends not on some critical and irreversible decision made in the process of differentiation, but on signals (likely neuregulin; Birchmeier and Nave (2008)) originating from its axon and related to its caliber (Weinberg and Spencer 1976; Aguayo et al. 1976; Pereira et al. 2012; Quintes et al. 2010). Axonal neuregulin signals information about axon size to Schwann cells; hypomyelination and hypermyelination result from reduced and overexpression of neuregulin, respectively (Michailov et al. 2004). Rarely, an unmyelinated axon develops one or more short myelinated internodes along its course, a phenomenon described particularly in aged animals (Heath 1982). Schwann cell biology has been the subject of a series of reviews collected in a special issue of Glia (Woodhoo and Sommer 2008).
5.1.1 Myelinating Schwann Cells
A myelinated fiber consists of an axon and its longitudinally arrayed series of SCs contained within a continuous basement membrane tube (the “Schwann tube”). Defined as that segment of the nerve fiber myelinated by a single SC, an internode ranges from 200 to 2,000 μm in length (Scherer 1999). The node of Ranvier represents the region where the territories of two Schwann cells meet and is an approximately 1 μm long axon segment not covered by myelin. If viewed longitudinally, the diameter of the myelinated fiber is much the same throughout the internode, excepting some swelling at the region of the nucleus and terminally at the paranodal region (Thomas et al. 1993). The nucleus of a myelinating SC is elongated and about 50 μm long, and thus, on cross sections about 3 % of large myelinated fibers and 9 % of small myelinated fibers are cut through the SC nucleus (Ochoa and Mair 1969).
5.1.1.1 Internodal Structure of Myelinated Fibers
Cross-sectional profiles at the internode reveal a compact myelin sheath surrounded at its internal (adaxonal) and external (abaxonal) aspects by thin rims of SC cytoplasm (Fig. 5.1). Conceptually, if the spiral myelin sheet is unrolled, most of it is seen to consist of compact myelin, with inner and outer semi-compact belts which contain thin layers of Schwann cell cytoplasm. The Schwann cell nucleus, elongated parallel to the axis of the fiber and found at mid-internode, is associated with an expansion of the cytoplasm where organelles such as elongated mitochondria, endoplasmic reticulum, a Golgi apparatus, 10 nm thick filaments, microtubules, the occasional centriole, and a number of inclusions can be identified. Variable amounts of free cytoplasmic glycogen are present. The vast majority of SC organelles are concentrated in the perinuclear region. Plasmalemmal vesicles (caveolae) fuse with the outermost layer of Schwann cell membrane in regions of cytoplasmic expansion (Mugnaini et al. 1977).
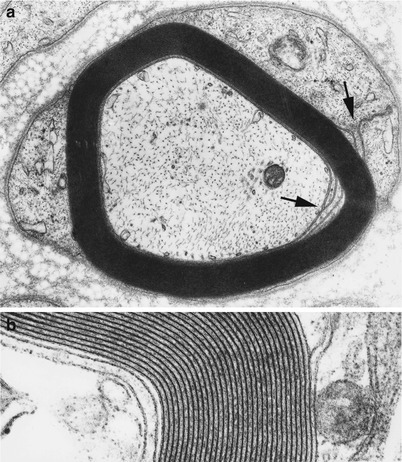
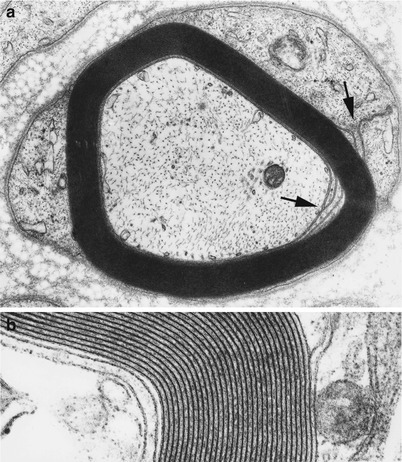
Fig. 5.1
Normal myelinated fiber. Note proximity of inner and outer mesaxons (arrows, a). High-magnification view allows resolution of the double layer of the intraperiod line (b) (a 32,200×; b 131,520×)
The first turn of the myelin sheath overlaps itself at the inner mesaxon. A tight junction seals this site of overlap and leads into the first intraperiod line, formed by the apposition of two outer faces of Schwann cell plasma membrane. Separating the innermost aspect of the Schwann cell sheath and the axolemma is an extracellular gap with a constant width of 12–14 nm. The outer mesaxon is the site where the last turn of Schwann cell cytoplasm overlaps itself and has a tight junction just before opening up to the extracellular space. A basement membrane invariably encloses the outermost portion of Schwann cell cytoplasm. This extracellular layer, composed of laminin, collagen types IV and V, heparan sulfate, and other macromolecules, forms a continuous “Schwann tube” around the nerve fiber, extending from cell body to nerve terminal (Bunge 1993). Cell culture studies have demonstrated that Schwann cells synthesize their own basement membrane (Bunge 1993).
5.1.1.2 Schmidt–Lanterman Clefts
The adaxonal and abaxonal semi-compacted and uncompacted Schwann cell cytoplasmic regions communicate through channels that run transversely across the spiraled compact myelin layers. These are the Schmidt–Lanterman (SL) clefts (Ghabriel and Allt 1981) (Figs. 5.2 and 5.3). Schmidt–Lanterman incisures are distinguished from compact myelin by the presence of Schwann cell cytoplasm (i.e., the major dense lines are not fused).
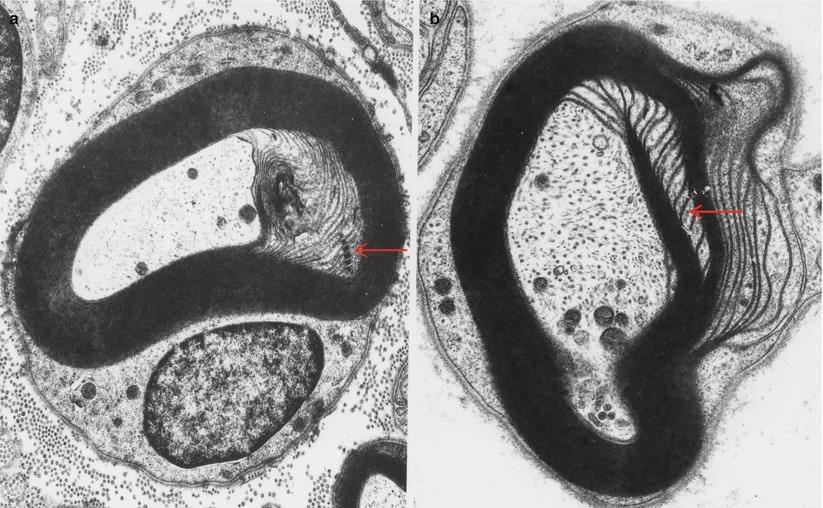
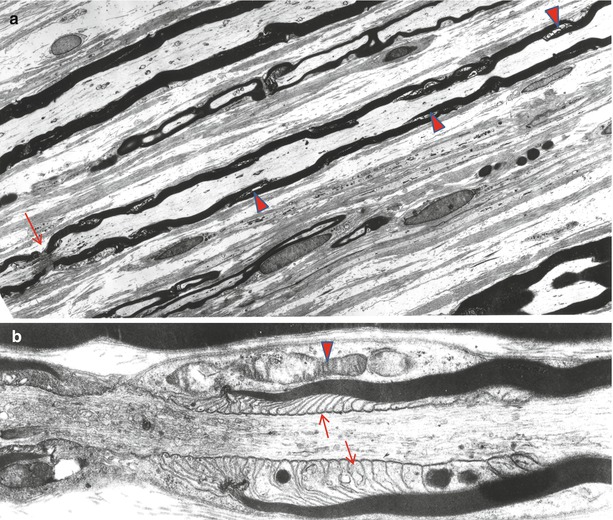
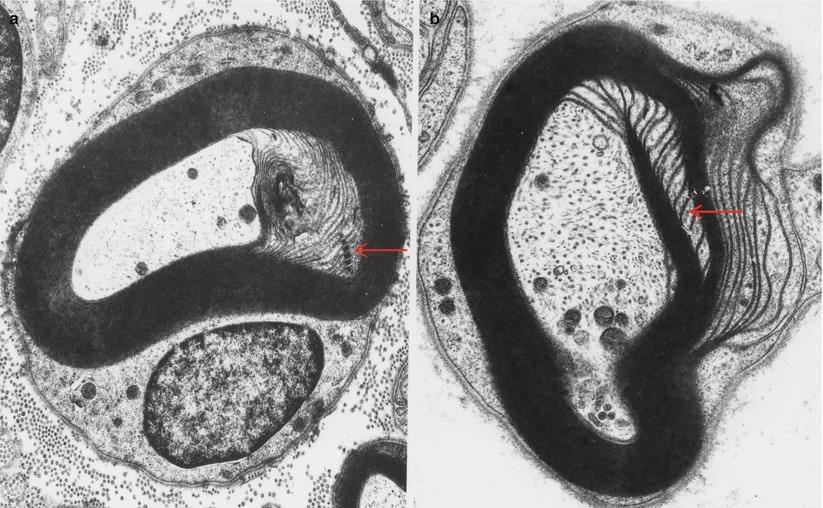
Fig. 5.2
Schmidt–Lanterman incisures. Note hemidesmosome-like areas of attachment in (a, arrow). Microtubules are present in the cytoplasm of the incisures (b, arrow) (a 13,307×; b 21,888×)
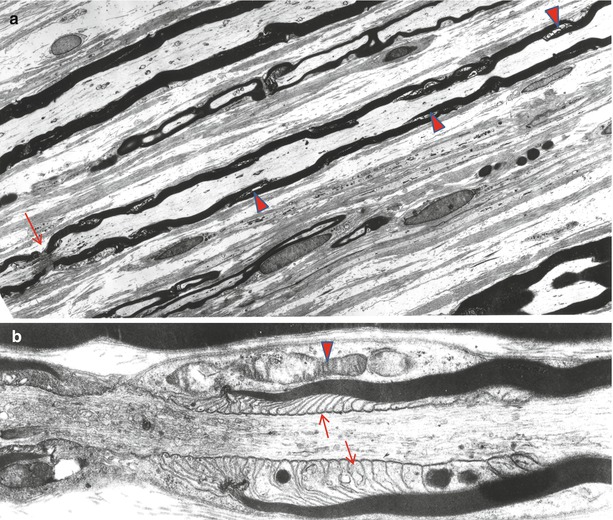
Fig. 5.3
Longitudinal view shows myelinated fibers and a node of Ranvier (arrow). Note that incisural cones do not have the same orientation (arrowheads). In (b) the paranodal area shows terminal cytoplasmic pockets (arrows) attaching to the axon and condensation of the nodal and paranodal axoplasm. The paranodal area displays a pocket of Schwann cell cytoplasm containing mitochondria (arrowhead) (a 1,450×; b 17,040×)
Because Schmidt–Lanterman clefts are fragile structures, a fully preserved cleft is rare in ordinary biopsy material. Typically, one sees a partial or full circumferential myelin “split” representing the disrupted SL cleft region (Fig. 7.1). When an intact SL cleft is seen in cross sections, it appears as partial or full circumferential array of stacked Schwann cell cytoplasmic pockets formed by focal separation of myelin at the major dense line (Fig. 5.2). On longitudinal cuts these pockets are revealed to be conically arranged and form an angle of about 9° to the long axis of the nerve fiber (Fig. 5.3a). The apex of the SL cleft “cone” does not point in a definite direction, and adjacent clefts may point in the same or opposite direction (Fig. 5.3a).
The linear density of SL clefts increases with the number of myelin lamellae present. In large myelinated fibers of human sural nerve, Buchthal et al. (1987) found about 35 clefts per mm, with each cleft having a length of about 13 μm. In small myelinated fibers of human sural nerve, these authors found about 8 clefts per mm of internode, each cleft measuring about 9 mm in length. Thus, with cross-sectional cuts one might expect to see clefts in about a 33–50 % of large myelinated fibers and 5–10 % of small myelinated fibers. Schmidt–Lanterman clefts can be seen in even the thinnest myelinated fibers (Hall and Williams 1970; Ghabriel and Allt 1981).
The cytoplasm within SL clefts often has a granular appearance and typically contains a single microtubule and less often membrane-bound organelles including mitochondria (Fig. 5.3). Incisure membranes are similar to paranodal loop membranes in that they lack compact myelin membrane proteins and contain myelin-associated glycoprotein (MAG). Immunohistochemical studies have shown preferential localization of connexin 32 to Schmidt–Lanterman incisures. The outermost few turns of the SL cleft may show a desmosome-like region of electron density. In addition to typical SL clefts, the myelin sheath contains some transverse cytoplasmic channels which terminate blindly and do not connect the inner and outer cytoplasmic belts. The myelin sheath also has longitudinal channels that run parallel to the axis of the nerve fiber (Mugnaini et al. 1977).
The function of SL clefts is unknown. They were previously considered by some to be artifactual, and indeed a distortion of the SL clefts is responsible for most myelin splits seen in cross sections (Fig. 7.1). However, in vivo studies conclusively prove their existence (Hall and Williams 1970). They may serve as a pathway of intracellular communication between the inner and outer Schwann cell cytoplasmic compartments. Some investigators have suggested that SL clefts give the myelin sheath a site into which newly synthesized cell membrane can be incorporated (Celio 1976) and may allow nerve fibers to tolerate bending and stretching better (Buchthal et al. 1987).
In Wallerian degeneration, early alterations occur at the SL clefts and the initial myelin ovoid consists of the myelin between two clefts (Williams and Hall 1971; Ghabriel and Allt 1979). In uncompacted myelin, a pathological alteration most often seen in paraproteinemic neuropathies with the POEMS syndrome, loss of myelin compaction, often begins at the Schmidt–Lanterman clefts; indeed it is sometimes difficult to distinguish between early loss of myelin compaction and normal variation in the form of the SL cleft (vide infra). Schroder and Himmelmann (1992) have studied pathological alterations at the SL cleft. Their work describes described granular degeneration, vesiculation, formation of membranous whorls, and accumulation of inclusions at the SL clefts as early but nonspecific findings in neuropathy, although more often seen in demyelinating processes.
5.1.1.3 The Nodal and Paranodal Structures
The paranodal segments are 5–7.5 μm in length at either side of the internode, depending on axon diameter (Berthold and Rydmark 1983). As the fiber approaches the node (Fig. 5.4a), it loses its rounded contour and develops grooves in both the myelin and the underlying axon, creating a crenated cross-sectional appearance (Fig. 5.4b). Within each crenation are cytoplasmic accumulations of membranous organelles; mitochondria are especially numerous (Figs. 5.3b and 5.4b). The appearance of the axon at this region is described in detail in Chap. 4. Further structural and molecular details regarding nodal and paranodal organization are provided in a variety of publications (Berthold and Rydmark 1983; Salzer et al. 2008; Scherer 1999; Scherer and Wrabetz 2008; Thomas et al. 1993; Buttermore et al. 2013; Kidd et al. 2013). The last 3–5 μm of the internode is the site of myelin loop termination. Seen longitudinally, the innermost myelin lamella terminates first, as the major dense line opens up to form a small “terminal cytoplasmic pocket.” This pocket attaches to the axolemma by a gap junction-like complex (Fig. 5.3b). The periaxonal space at this point of attachment measures 3–5 nm, in contrast with the 12–14 nm seen in the internodal region. The juncture of myelin loops with the underlying axolemma is a complex arrangement involving contactin, CASPR, and an isoform of neurofascin, NF155 (see Kidd et al. for review). Subsequent lamellae behave similarly, but in thickly myelinated axons there is insufficient room for all the lamellae to attach to the axons, so 80–90 % of the terminal myelin loops are coiled on top of one another in sets of 10–25, creating a picture reminiscent of “ears of barley” (Thomas et al. 1993). Junctions attach terminal myelin loops to one another and to the axolemma where possible. Freeze fracture studies reveal a complex membrane particle structure, some of which represents ion channels essential for the electrical conducting function of the axon (Wiley and Ellisman 1980).
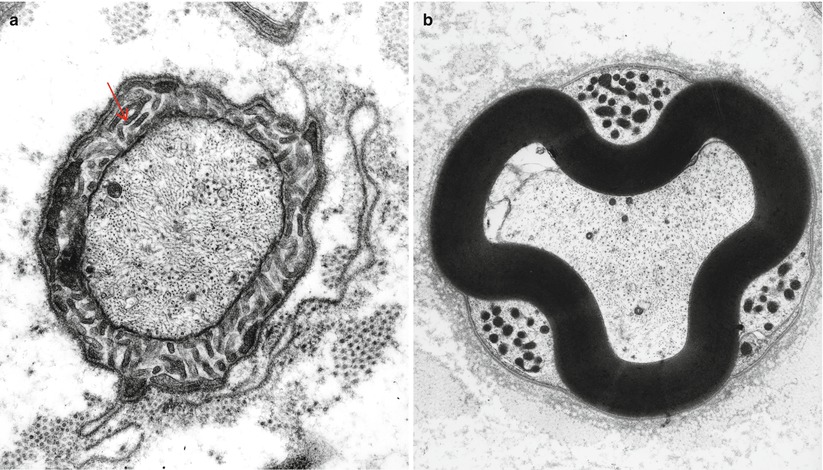
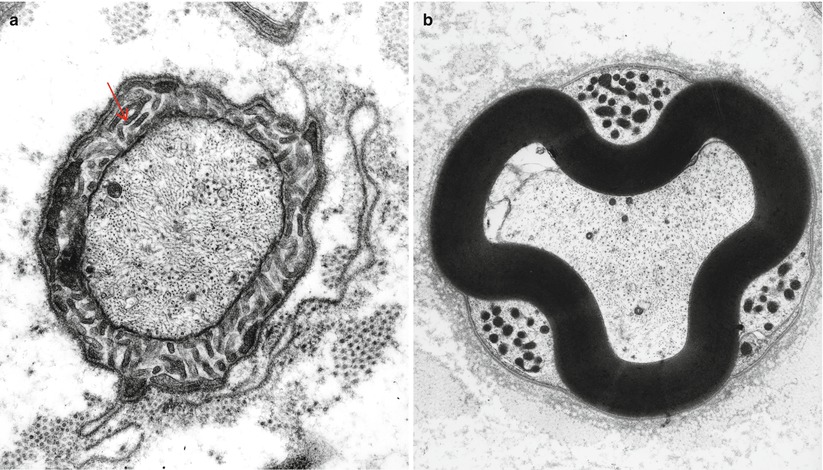
Fig. 5.4
Cross section through the node of Ranvier (a) and the paranodal area (b). In (a) Schwann cell microvilli (arrow) surround the axon. In (b) the crenated appearance of myelin conforms to three mitochondria containing pockets (a 16,302×; b 9,230×)
A space of about 1 μm separates the terminal myelin loops of one paranode from those of the one opposing it. Through the center of this space runs the axon (Fig. 5.3b). Slightly overlapping extensions of the Schwann cell of each internode roof over the nodal space. These extensions project numerous radially arrayed microvilli into the nodal gap, to terminate within 5 nm of the axon (Fig. 5.4a). There is a gap substance around microvilli filling the space between the Schwann cell nodal processes and the basal lamina. This gap substance contains glycosaminoglycans with cation-binding and exchange properties maintaining an osmotically inactive concentration of sodium ions and limiting diffusion of K+ away from the node. The microvilli are enriched in actin filaments and actin-associated proteins. The Schwann tube (basal lamina) that surrounds all Schwann cells bridges over the nodal and paranodal structures and has equally complex constituents consisting of collagens, laminins, nidogen (entactin), fibronectin, and proteoglycans such as glypican and perlecan (Aszodi et al. 2006).
5.1.2 Myelin
Myelin is a multilamellated membranous substance produced through structural and biochemical modification of Schwann cell plasma membrane (reviewed in Webster 1993; Mezei 1993; Scherer 1999; Scherer and Wrabetz 2008; Svaren and Meijer 2008). Schematic drawings invariably illustrate mature myelin as a smooth cylindrical structure, but in reality serial sections reveal occasional infoldings and outpouchings, which in cross sections may appear as isolated “ovoids” or “loops” of myelin. Such alteration is more common in large myelinated axons, particularly near the node (Webster and Spiro 1960). The appearance can be misinterpreted as artifactual or as an indicator of pathology, as emphasized by Webster and Spiro (1960). Elzholz bodies, and myelin “wrinkling,” are probably related to this myelin irregularity.
5.1.2.1 Determinants of Internode Parameters
The three principal parameters that govern conduction in a myelinated fiber are axonal diameter, myelin thickness, and internode length (Waxman 1980). Maximal internodal lengths are about 200–300 μm at birth and increase to adult values in close parallel with somatic growth (Gutrecht and Dyck 1970; Schlaepfer and Myers 1973; Friede et al. 1981). The actual site of a node of Ranvier is determined prior to the onset of myelination by specializations of the axolemma which presumably interact with the Schwann cell to limit its longitudinal growth (Wiley-Livingston and Ellisman 1980; Waxman 1980). Somatic elongation from onset of myelination to adult life is the main factor governing internode length (Friede et al. 1981). Larger-diameter axons have longer internodes, perhaps because these fibers myelinate earlier and are thus subject to a relatively greater degree of somatic growth (Thomas and Young 1949; Schlaepfer and Myers 1973). However, this explanation is incomplete, as the total complement of large and small myelinated fibers in human nerve is probably obtained very early in life (Jacobs 1988).
Myelin thickness is proportional to axon diameter, and in a first approximation, a single linear relationship provides a good degree of correlation across all fiber sizes (Behse 1990). However, small myelinated fibers are relatively more thickly myelinated than large myelinated fibers (Behse 1990; Friede and Bischhausen 1982). For axons of a given diameter, myelin thickness seems to increase slightly with internode length, a finding that is congruent with theoretical considerations (Friede and Bischhausen 1982; Friede and Beuche 1985).
5.1.2.2 The Structure of Compact Myelin
The periodicity of compact PNS myelin in vivo is 18 nm, although fixation usually reduces this to 15 nm, mostly through dehydration (Kirshner and Ganser 1984). The major dense line is about 6 nm thick and is made up of the fused cytoplasmic aspects of the Schwann cell membrane. In vivo the major dense line is actually composed of two membrane leaflets separated by about 2 nm of intracellular space. However, glutaraldehyde fixation and osmium tetroxide postfixation remove water and some membrane lipids, resulting in fusion of the two layers (Kirshner and Ganser 1984). The intraperiod line (IPL) is made up of two thinner lines, each representing the outer leaf of the Schwann cell plasma membrane, separated by about 4 nm (Fig. 5.1b). Thus, the region between the two leaves of the IPL is contiguous with the extracellular environment, and the space within the major dense line (in vivo) is contiguous with the Schwann cell cytoplasm.
Although the space within the IPL is contiguous with the extracellular matrix, junctional complexes at the outer and inner mesaxons and at the nodal area somewhat restrict flow of molecules and ions in this space (Chernousov et al. 2008). Because the cell membranes lining the IPL space have a negative charge (Inouye and Kirschner 1988), cations find their way into it more readily than anions or neutral molecules (Ropte et al. 1990). The presence of cations within this space is important for maintenance of the normal periodicity, as removal of positive charges results in widening of the space, presumably due to repulsion from unmasked opposing negative charges (Ropte et al. 1990).
5.1.2.3 Composition of Myelin
Myelin is a modification of the Schwann cell membrane. It contains 45 % water by weight, and the composition of dehydrated human myelin is 71 % lipid and 29 % protein (Inouye and Kirschner 1988; Norton and Cammer 1984). The important lipid components are phospholipids (55 % of total lipids), glycolipids (cerebrosides, sulfatides, and gangliosides representing 22 % of total lipid), and cholesterol (23 % of total lipid). Physiologically and clinically important proteins include P0, P1, and P2 glycoproteins, peripheral myelin protein (PMP)-22, and myelin-associated glycoprotein (MAG).
Glycolipids occur only on the external layer of the Schwann cell membrane and thus are localized to the intraperiod line (Inouye and Kirschner 1988). Immunization of experimental animals with galactosylceramide can produce experimental allergic neuritis (EAN), an animal model for Guillain–Barré syndrome (Stoll et al. 1986). Monoclonal and polyclonal antibodies to gangliosides have been identified as potential causative agents of human demyelinating and axonal neuropathies. Metachromatic leukodystrophy and Krabbe leukodystrophy are inherited diseases with defects in glycolipid metabolism which produce a demyelinating neuropathy, although the mechanism of the neuropathy is uncertain.
P0 is a 30 kDa transmembrane glycoprotein which makes up about 60 % of all PNS myelin protein by weight, but is not present in CNS myelin or in nonmyelinating Schwann cells (Giese et al. 1992). It may function in maintaining the compaction of myelin at both the intraperiod and major dense lines (Lemke et al. 1988; Filbin and Tennekoon 1991). Immunization with P0 protein can produce EAN (Milner et al. 1989), and antibody against P0 protein has been associated with a severe childhood hypertrophic neuropathy (Ben Jelloun-Dellagi et al. 1992). Mice with defective P0 protein expression show loss of most myelin compaction. However, some degree of myelin organization remains, indicating that proteins other than P0 are important in this role (Giese et al. 1992). Mutations in P0 protein have been implicated in the human familial neuropathies CMT-1B and Dejerine–Sottas syndrome (CMT-3).
P1 protein (identical to CNS myelin basic protein) has been extensively studied in its role as the major neuritogenic antigen of experimental allergic encephalitis (Linington and Brostoff 1993). This is a glycoprotein with multiple isoforms, of which the 18 kDa variant is the most important. It accounts for a species variable 2–16 % of PNS protein. P1 is found on the cytoplasmic aspect of the Schwann cell membrane, but its function in PNS myelin is largely unknown (Linington and Brostoff 1993; Peterson and Bray 1984).
P2 is a 14.8 kDa glycoprotein found predominantly in the PNS where it makes up 2–15 % of myelin protein (Linington and Brostoff 1993). It is located in the cytoplasmic layer of Schwann cell membrane, particularly in regions where myelin is not compacted (Trapp et al. 1979). The physiologic function of P2 protein is uncertain. P2 glycoprotein is the major antigenic stimulus for production of EAN by immunization with peripheral nerve myelin (Rostami 1993).
Peripheral myelin protein-22 (PMP-22) is a more recently discovered 18 kDa integral membrane protein found primarily in PNS myelin (Suter et al. 1993). Although it is localized to compact myelin, its physiologic importance is unknown. Mutations in the PMP-22 gene in mice produce the Trembler phenotype. In humans, there is a strong association between defects involving the PMP-22 gene and CMT-1A hypertrophic neuropathy as well as hereditary neuropathy with liability to pressure palsies (HNPP).
Myelin-associated glycoprotein (MAG) is a 100 kDa transmembrane protein found in both the CNS and the PNS. This protein has an immunoglobulin-like domain on the outer face of the Schwann cell/oligodendrocyte membrane. Quantitatively, it makes up only 0.1 % of PNS myelin protein (Trapp 1990). In PNS myelin, MAG is found in the periaxonal space, paranodal regions, Schmidt–Lanterman clefts, and inner and outer mesaxonal membranes (Trapp 1990; Kidd et al. 2013). All membranes containing MAG appose other membranes by 12–14 nm (Trapp 1990). MAG is a member of the family of cell adhesion molecules and may play a role in maintaining the anatomical and physiologic integrity of the myelin sheath–axon unit. It may also be important in initiating the process of myelin formation and in controlling which parts of the Schwann cell cytoplasm become compacted (Leblanc and Poduslo 1990; Trapp 1990). IgM paraproteins with specificity against MAG have been strongly implicated in the causation of a common human demyelinating neuropathy.
5.1.3 Nonmyelinating Schwann cells
In humans sural nerve nuclear counts show that nonmyelinating Schwann cells (NMSCs) outnumber myelinating SCs by a factor of 4:1 (Ochoa and Mair 1969). Significant differences exist in the biology of nonmyelinating Schwann cells compared to myelinating Schwann cells (reviewed in detail by Griffin and Thompson 2008). In contrast to the strict 1:1 relationship between axons and Schwann cells seen in myelinated fibers, single or multiple NMSC profiles may be seen without associated axons, or a single NMSC may be seen to support one, two, or more axons. A Schwann cell subunit (ScSu) has been defined as any single Schwann cell profile or group of profiles that in cross section is enclosed by a continuous basal lamina (Sharma and Thomas 1975). Schwann cells surrounding a collection of unmyelinated axons have been designated as Remak fibers, a term which refers only to unmyelinated fibers. Remak Schwann cells have territories that extend longitudinally for 50–100 μm and ensheathe different axon modalities. Unmyelinated axons exchange frequently among different Remak bundles during their proximodistal courses (Kidd et al. 2013). Quantitative aspects of Schwann cell subunits are reviewed elsewhere. The discussion here will focus on ultrastructural features.
Schwann cell cytoplasm completely encircles most unmyelinated axons, although occasionally one may see a segment of axon that is in direct contact with the surrounding basement membrane. The formation of individual mesaxons for each unmyelinated axon is thought to be the result of secretion of neuregulin 1 type III (Taveggia et al. 2005). Nonmyelinating Schwann cell cytoplasm contains the same complement of organelles as myelinating Schwann cells. The NMSC nucleus is elongated, 10–20 μm long, with only about 1 in 14 Schwann cell profiles showing the nucleus in cross section (Carlsen et al. 1974); the typical length of an NMSC has been estimated at 200–500 μm (Carlsen et al. 1974).
It may be difficult or impossible to distinguish a circular NMSC profile from an axon when the cross section contains no nucleus. Some criteria assist in making the distinction (Dyck 1969; Gibbels 1989). Axons are more likely to be round and tend to be less electron dense than Schwann cell processes. Neurofilaments and neurotubules are found in roughly equivalent numbers in unmyelinated axons, but tubules are far less prominent than filaments in Schwann cells. The formation of a mesaxon around a circular profile favors the possibility that the circular profile is an axon. The axolemma tends to have a greater electron density than the Schwann cell plasma membrane.
Occasionally, several Schwann cell processes may surround an axon. This occurrence usually relates to regions where the longitudinal extent of one Schwann cell ends and the next begins. These junctional points are not as clearly demarcated as the nodes of Ranvier in myelinated fibers. Studies by Eames and Gamble (1970) demonstrate how Schwann cell processes form variably complex regions of overlap and interdigitation at the junction point. Often the cytoplasm of the innermost Schwann cell at the site of overlap is particularly electron dense (Eames and Gamble 1970). Bundles of collagen may be partially or fully encircled by Schwann cell cytoplasm. These “collagen pockets” (Fig. 2.12) increase in prominence with age and in neuropathy. Denervated ScSus are identified by their lack of axons. This condition also occurs in normals, but increases with age or pathology.
5.1.3.1 Control of Myelination Phenotype
Myelination requires a balance of transcriptional programs (reviewed in Mirsky et al. 2008) with positive regulators of this process, including Krox-20, Sox-10, and Oct-6, and negative regulators (Notch, Sox-2, Pax-3, Id2, Krox-24, and Egr-3) which are expressed prior to myelination, downregulated as myelination starts, and reactivated as Schwann cells dedifferentiate following injury which is required for effective nerve regeneration.
5.1.4 Schwann Cell Inclusions
5.1.4.1 Reich Pi Granules
These inclusion bodies are typically found adjacent to the nucleus of myelinating Schwann cells (arrows, Fig. 5.5a, b). They can be seen by light microscopy and may number in the dozens within a perinuclear region (Fig. 5.6a, b). Reich identified their metachromasia with aniline dyes and noted that they had different staining properties than mast cell granules – a point of controversy at the time (Reich 1903). Pi granules are metachromatic with toluidine blue (Fig. 5.5a), Hirsch–Peiffer, and methylene blue stains, are refractile under polarized light (Fig. 5.5b), and will stain positively with Sudan black and PAS on frozen material (Noback 1953; Olsson and Sourander 1969). Their positive staining for acid phosphatase identifies them as lysosomes (Weller and Herzog 1970). Pi granules are best seen with the electron microscope, where they appear as single membrane-bound organelles, typically 1 μm in size, whose contents are often partially or totally removed in processing. However, when a structure can be discerned, there is usually a lamellar pattern with a periodicity less than that of myelin (5 nm vs. 12–17 nm) (Fig. 5.6b). At time they may take a “zebra body”-like appearance or contain globules of amorphous variably osmiophilic material. Their shape may be elongated, oval, or polygonal. In nerves undergoing active degeneration, we have seen pi granule-like inclusions within macrophages, perhaps representing the indigestible remains of Schwann cells. Thomas et al. (1993) have indicated that pi granules are not found in nonmyelinating Schwann cells. Although pi granule-like inclusions can rarely be seen in NMSCs, such configurations may represent denervated, formerly myelinating, Schwann cells.
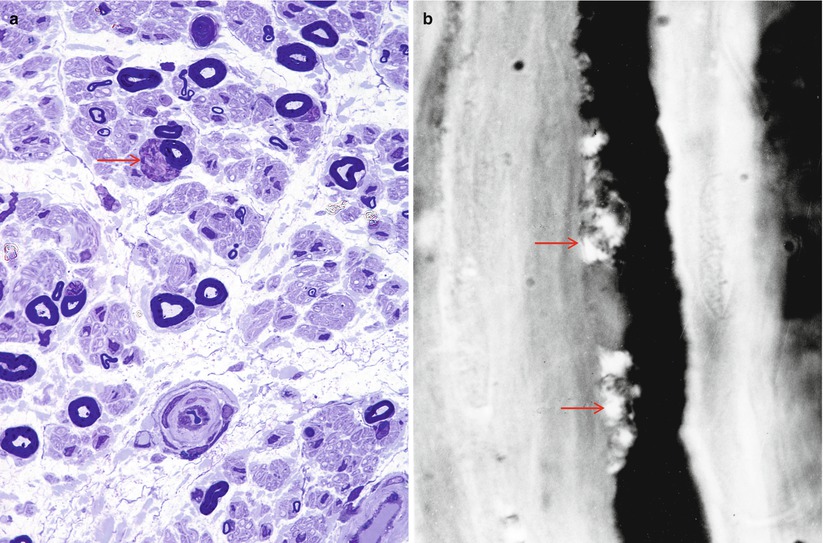
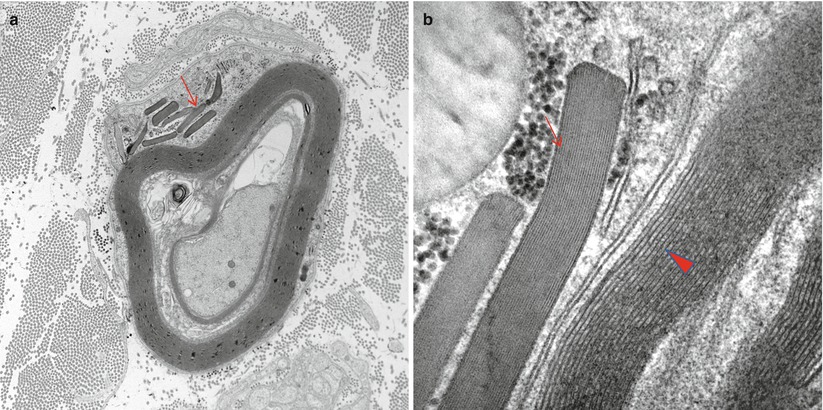
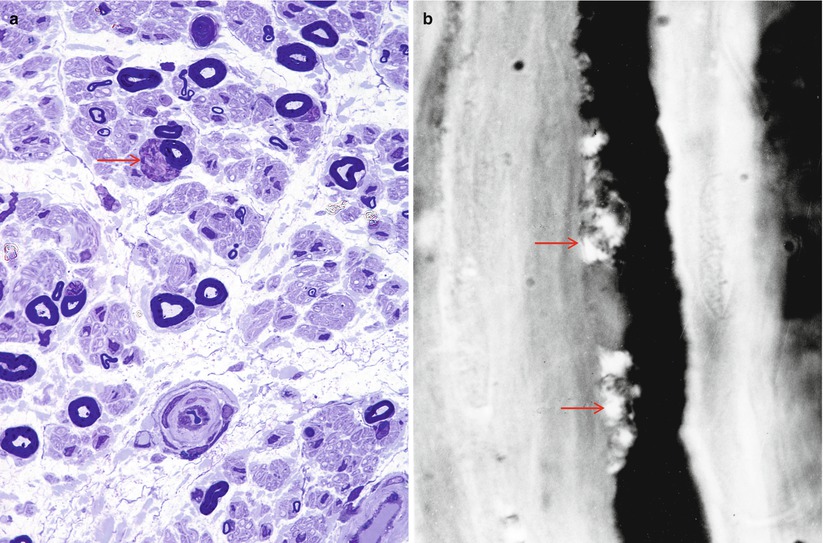
Fig. 5.5
Reich pi granules. These osmiophilic and metachromatic structures (arrow, a) are usually found in the perinuclear Schwann cell cytoplasm. In (b) a teased fiber preparation demonstrates refractile pi bodies (arrows) adjacent to the nucleus (a 1 µ plastic, 1,000×; b teased fiber photographed under half-crossed polarizing filters)
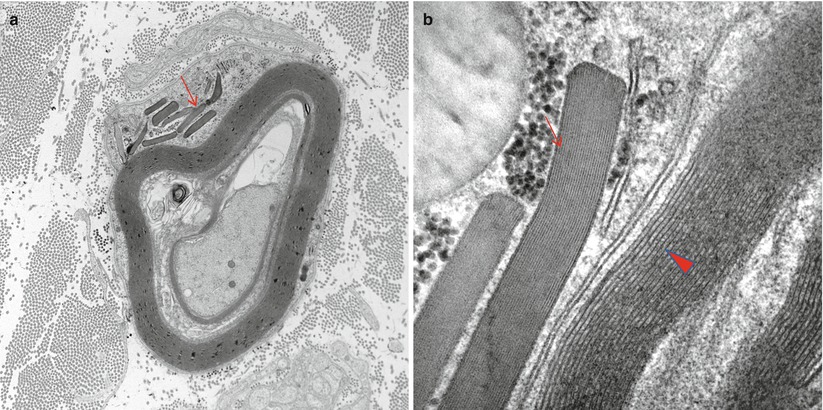
Fig. 5.6
Reich pi granules. Membrane-bound pleomorphic inclusions of variable osmiophilia are seen in the perinuclear region. Some rodlike granules (arrow, a) have a fine periodicity different from adjacent myelin (arrowhead, b) (a 12,000×; b 100,000×)
Reich pi granules normally appear in myelinating Schwann cells, but are mostly restricted to the perinuclear area, so most fibers seen in cross sections do not show them. Rarely, they can be encountered in the paranodal Schwann cell cytoplasm. They are said to not be present in nerves of young people (<8 years of age, Robson 1951); Reich himself (1903) pointed out that the inclusions were not seen in the first years of life. Most authors have indicated, without formal quantitation, that pi granule numbers increase with age, suggesting that they are a “wear-and-tear”-related accumulation (Babel et al. 1970, p 48; Weller and Herzog 1970; Leibowitz et al. 1983; Asbury and Johnson 1978; Thomas et al. 1980; Robson 1951). It is noteworthy that they are not seen in recently remyelinated fibers (Weller and Herzog 1970).
Pi granules can be more prominent in neuropathy (Evans et al. 1965; Dyck and Lambert 1970; Shetty et al. 1988), but this is nonspecific. They may also be seen in increased numbers in some storage diseases (Goebel et al. 1976), but workers should exercise care not to confuse them with zebra bodies or other abnormal storage material (Olsson and Sourander 1969).
5.1.4.2 Elzholz Bodies (Mu Granules)
Elzholz bodies are rounded, osmiophilic, Marchi-positive bodies containing unsaturated lipid seen in the cytoplasm of myelinating Schwann cells, external to compact myelin, and most frequently in the perinuclear and paranodal regions (Schroder and Himmelmann 1992; Dyck et al. 1984a) (Fig. 5.7). Electron microscopy reveals a periodicity similar to that of myelin. No pathological significance has been attached to Elzholz bodies, and they may be seen in normal nerves. Most likely their formation is related to myelin remodeling and to the loops and redundant myelin folds that are seen in normal and abnormal myelinated fibers (Webster and Spiro 1960; Schroder and Himmelmann 1992).
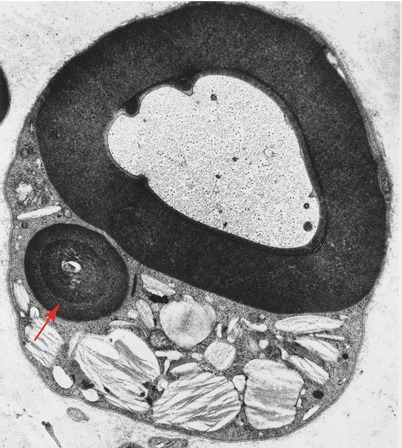
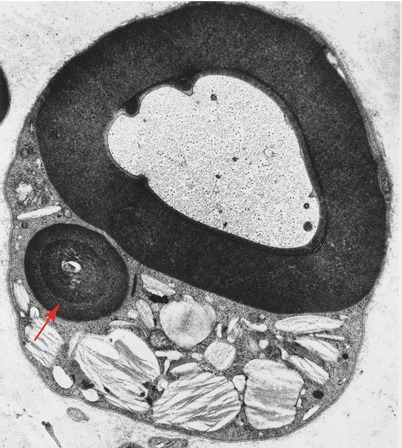
Fig. 5.7
Elzholz body. In contrast to pi bodies, the Elzholz body (arrow) is rounded and has the periodicity of myelin (11,400×)
5.1.4.3 Lipofuscin
Lipofuscin is an insoluble intracellular pigmentary accumulation seen in a variety of tissues throughout the body. Intracellular storage of lipofuscin results from the gradual accumulation of indigestible residues of autophagy and likely reflects the “wear and tear” of aging. In the PNS, the main site of accumulation of lipofuscin is in nonmyelinating Schwann cells, where the substance appears as autofluorescent single membrane-bound material, most of which is electron-dense amorphous or granular, intermingled with lightly or moderately electron-dense lipid droplets (Fig. 5.8). In one study the number of lipofuscin granules increased by a factor of 4 from age 17 to age 69 in unmyelinated fibers of human vagus nerves (Sharma and Thomas 1975). Although lipofuscin is normally not demonstrable in myelinating Schwann cells (Thomas 1993), such occurrence has been noted in Refsum disease (Thomas 1993), adrenoleukodystrophy, and Niemann–Pick disease. We have also examined a biopsy from a patient with Dejerine–Sottas syndrome in which myelinating Schwann cells contained abundant lipofuscin.
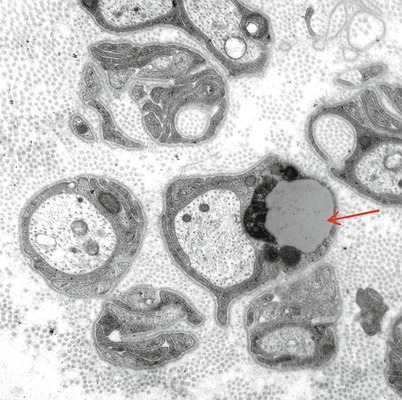
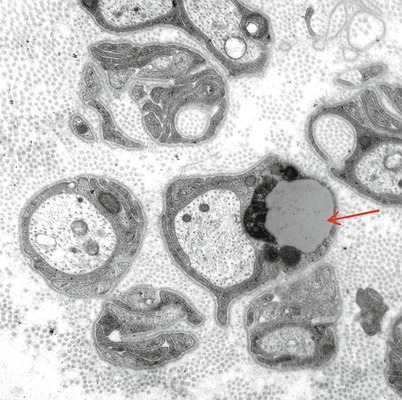
Fig. 5.8
Schwann cell inclusions. Lipofuscin (arrow) is ordinarily only found in nonmyelinating Schwann cells (magnification, a 20,235×; b 8,915×)
5.1.4.4 Other Inclusions
Some Schwann cell inclusions are of great value in the diagnosis of storage diseases and certain intoxications; these are discussed elsewhere (Table 7.9; Chap. 20). Other dense membrane-bound inclusions of uncertain significance may be seen in Schwann cells. Some of these probably would have been identified as “Elzholz bodies” by some workers. Osmiophilic inclusions seem to be more numerous in “sick” or stressed Schwann cells and probably represent autophagic vacuoles. Glycogen accumulation in the occasional myelinating and nonmyelinating Schwann cell can be striking, but is nonspecific. Increased Schwann cell glycogen may be particularly prominent in glycogenoses and hypothyroidism (Dyck and Lambert 1970).
One may observe double membrane-bound paracrystalline inclusions with small amorphous electron-dense regions in nonmyelinating Schwann cells (Fig. 5.9a, b). Such inclusions were initially emphasized in Refsum disease in which the authors hypothesized that they might be modified mitochondria (Fardeau and Engel 1969). Subsequently, workers identified similar inclusions in a host of toxic, metabolic, and genetically based neuropathies (Thomas and King 1974; Lyon and Evrard 1970; Vallat et al. 1973; Schroder and Sommer 1991). These inclusions have no diagnostic specificity and were found in 25 of 280 unselected peripheral neuropathies, in up to 10 % of NMSC mitochondria (Schroder and Sommer 1991). We have observed such inclusions in situations including CIDP, sarcoid neuropathy, disulfiram neuropathy, diabetic neuropathy, idiopathic axonal neuropathies, and a nerve showing only minimal changes attributable to aging. Similar inclusions have also been emphasized in mitochondrial diseases (Yiannikas et al. 1986; Schroder and Sommer 1991), may be very prominent in a variety of cell types in amiodarone neuropathy, and can be produced experimentally by treating rats with inhibitors of cholesterol synthesis (Hedley-White 1973; Suzuki and DePaul 1972).
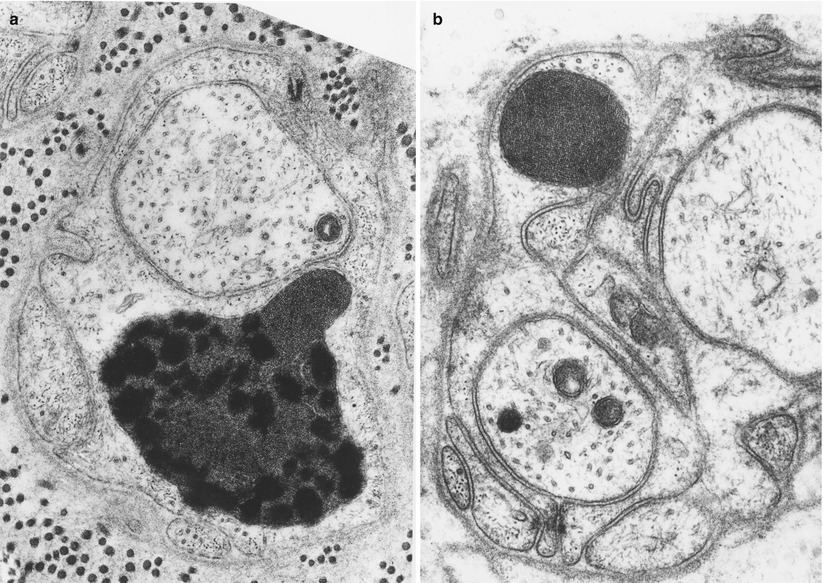
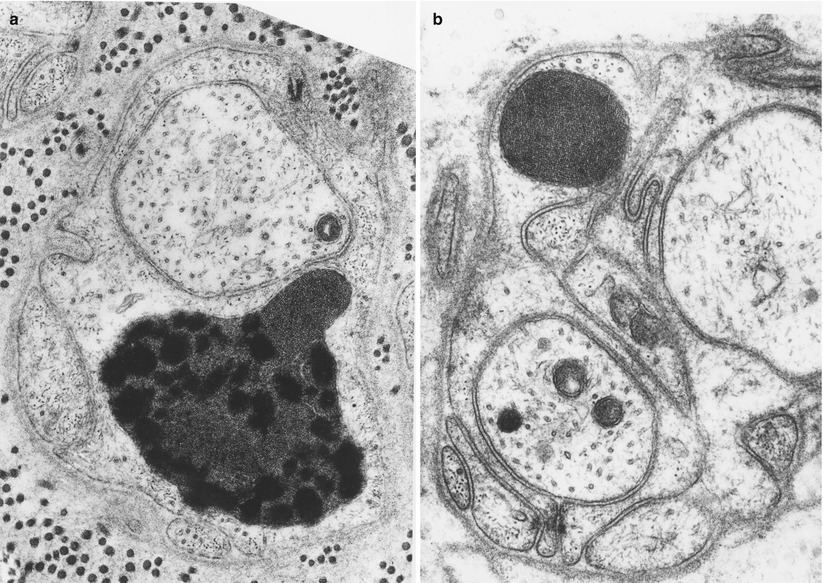
Fig. 5.9
Schwann cell inclusions. Paracrystalline inclusions are not uncommon in chronic neuropathies. These are probably of mitochondrial origin and may contain lipid (a 34,884×; b 35,700×)
5.2 Demyelination
When studying the pathology of a neuropathic disease, one must decide whether the process is fundamentally axonal or demyelinating, as this is of great diagnostic and prognostic importance. The distinction may not be obvious, as the Schwann cell and axon are highly interdependent; a diseased axon results in disturbance of Schwann cell metabolism and myelin structure, and myelin alterations affect axon morphology. Processes involving the nerve (toxins, trauma, ischemia, inflammation) seldom damage only myelin or only the axon.
Demyelination may occur as a consequence of Schwann cell or myelin defects, in which case it is primary demyelination. However, the typical changes of demyelination can also be seen in diseases known to primarily act upon the axon. This is termed secondary demyelination. Primary demyelination may be a consequence of Schwann cell dysmetabolism with loss of ability to maintain the myelin sheath or may result from direct attack on myelin itself. The processes leading to myelin damage are many, but often the appearance of the resultant demyelination is nonspecific. Below, we review both nonspecific and specific features of demyelination with emphasis on elements of differential diagnosis.
5.2.1 Nonspecific Features of Demyelination
A normal large diameter axon either surrounded by degenerating myelin or entirely denuded constitutes clear-cut light microscopic evidence of ongoing demyelination (Figs. 5.10a, b and 5.11). Myelin debris, consisting of osmiophilic material contained in cells around the axon or elsewhere in the endoneurium, indicates that the process is actively proceeding, as exogenous phagocytes clear debris within days to weeks. Although Schwann cells are capable of taking up myelin debris in nonphysiologic situations, they seem to leave this task to macrophages in vivo (Friede and Bruch 1993; Griffin et al. 1993).
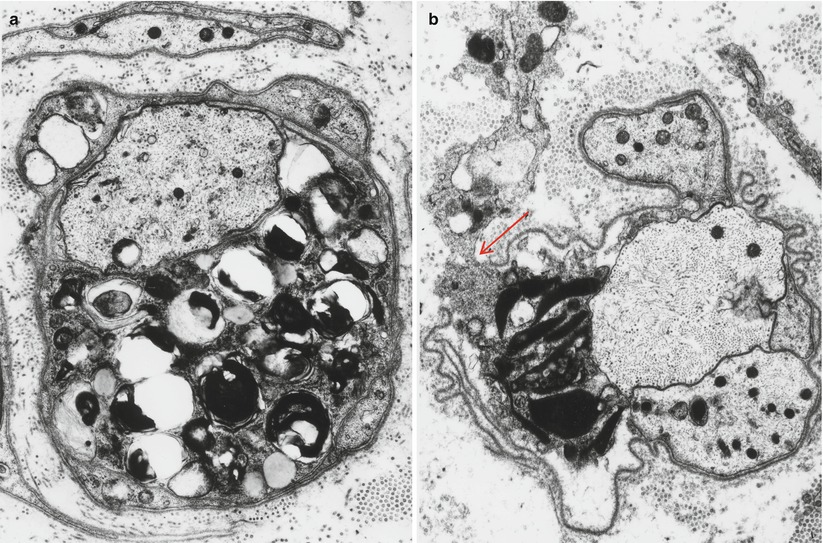
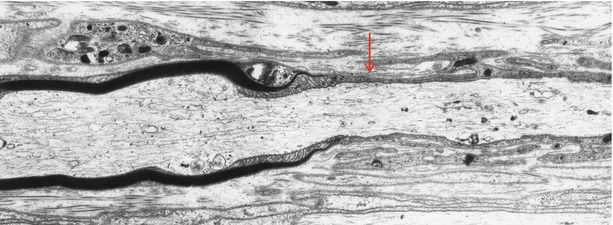
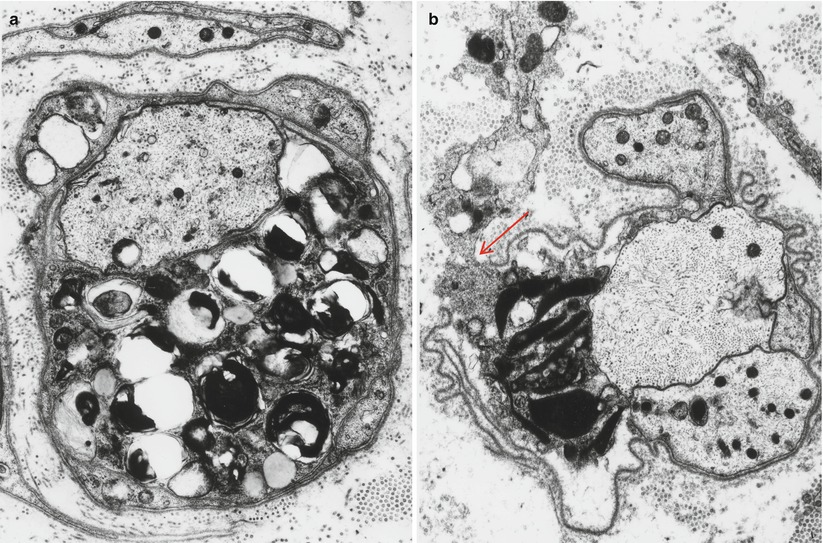
Fig. 5.10
Demyelination. In (a) only one of the intratubal cell processes contains debris and likely belongs to a macrophage. In (b) a macrophage process is captured exiting through a wide gap in the basal lamina (arrow) (a 13,760×; b 12,070×)
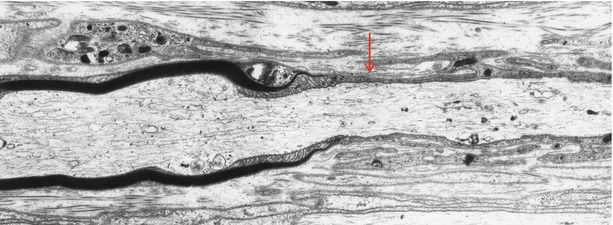
Fig. 5.11
Demyelination. Note interface (arrow) between remyelinated internode and a demyelinated axon (7,800×)
Early myelin changes can include splitting or vacuolation of the compact myelin sheath, focal areas of vesicular degeneration, and the appearance of myeloid bodies in Schwann cell cytoplasm. Alterations in terminal myelin loops at the node of Ranvier, small myelin “ovoids” in the paranodal regions, vesicular degeneration, and retraction of Schwann cell processes with resultant widening of the nodal gap are considered early changes in demyelination of all varieties (Asbury et al. 1969, 1978; Lampert and Schochet 1968; Masurovsky et al. 1967; Allt 1983), including that secondary to axonal disease (Dyck et al. 1981), and in axonal degeneration (Ballin and Thomas 1969a). Thus, paranodal myelin alterations should not be interpreted as evidence of a primary demyelinating process.
While a degenerating or absent myelin sheath around an intact formerly myelinated axon directly indicates demyelination, a thinly myelinated axon is a sign of remyelination. The hallmark of recurrent demyelination and remyelination is onion-bulb formation, consisting of a central axon (myelinated or otherwise) surrounded by concentric attenuated Schwann cell lamellae. Onion bulbs are described and illustrated elsewhere, and remyelination is considered below.
Buckling and splitting of myelin, inpouching and outpouching of myelin folds, and focal separation of the axon from its myelin sheath are commonly observed in nerve biopsies. Such changes can be seen in otherwise normal nerves but are clearly more prominent in neuropathy and correspond to myelin “wrinkling” seen on teased fibers (Dyck et al. 1971, 1984b; Webster and Spiro 1960). This alteration increases in frequency with the normal aging process (Dyck et al. 1993, Table 30.4) and is particularly common with myelin remodeling in response to axonal atrophy (Dyck et al. 1984b). Mechanical or chemical trauma during the biopsy procedure might also produce some of these alterations.
5.2.2 Specific Myelin Changes in Primary Demyelinating Neuropathies
5.2.2.1 Macrophage-Mediated Myelin Stripping
Destructive stripping of myelin by macrophages is the central mechanism of demyelination in the inflammatory demyelinating neuropathies, GBS and CIDP, and is generally regarded as specific to these entities (Prineas 1981; Brechenmacher et al. 1987). The macrophage penetrates the Schwann cell basement membrane and the cell membrane, separates Schwann cell cytoplasm from its myelin, and insinuates cytoplasmic fingers into the intraperiod lines of the myelin sheath. Most often this occurs in idiopathic CIDP and GBS; however, macrophage-mediated demyelination is also seen when these clinical syndromes occur in the setting of other illnesses, such as HIV infection (Cornblath et al. 1987), a circulating IgG (Pollard et al. 1983; Bleasel et al. 1993) or IgM (Vital et al. 1991) paraprotein, or lymphoma (Sumi et al. 1983). Macrophage-mediated demyelination is seen in EAN, the animal model of GBS (Wisniewski et al. 1969), and several other veterinary diseases (Marek’s disease, coonhound paralysis). It also appears in recurrent lysophosphatidylcholine-induced demyelination, probably as a consequence of “self-immunization” against myelin following repeated episodes of iatrogenic demyelination (Hall 1984). Macrophage-mediated myelin stripping has rarely been described in familial hypertrophic neuropathy (Vital et al. 1992; Madrid et al. 1977) or uremia (Said et al. 1983), but we have never observed this and find the illustrative figures to be unconvincing, perhaps simply showing scavenger macrophages which have entered the Schwann tube to clear out degenerating myelin. An alternative interpretation is that these cases represent instances of inflammatory demyelinating neuropathy superimposed on another neuropathy.
Macrophage-mediated demyelination is likely the final common pathway of a variety of dysimmune phenomena. Complement and immunoglobulin deposits have been found on the Schwann cell membrane in a variety of inflammatory neuropathies (Hays et al. 1988). In theory, these substances can opsonize myelin and promote phagocytosis via the immunoglobulin and complement receptors normally found on macrophages (Stoll et al. 1991). Indeed, intact myelin seemed to be taken into coated pits in a study of inflammatory demyelination in the CNS (Epstein et al. 1983), and this process may be initiated by IgG binding to the myelin sheath (Wayne Moore and Raine 1988). However, the finding of myelin-binding antibodies or complement has been inconsistent in both CIDP and GBS (see discussion Chap. 9), and these deposits may be an epiphenomenon relating to the alteration in vascular permeability often seen early in the course of inflammatory neuropathy.
Investigators generally regard the insertion of cell processes and removal of myelin debris as discussed above and in more detail elsewhere as unique to macrophages. However, Schwann cells of unmyelinated fibers displayed identical behavior when serum from GBS patients was introduced into a neural cell culture line in a macrophage-free medium (Birchem et al. 1987). There are also reports of malignant lymphocytes in CLL apparently carrying out myelin stripping (Vital et al. 1975; Sumi et al. 1983).
5.2.2.2 Vesicular Demyelination
Myelin can sometimes disintegrate around an apparently intact axon through splitting at the major dense line, creating a netlike pattern of vesicles 80 nm in diameter (see Fig. 7.4a-c) (Arnason and Soliven 1993; Brechenmacher et al. 1987). In a different plane of section, these are seen as parallel lines, suggesting that in three dimensions the structure is composed of parallel cylinders (Carpenter 1972). Detached pieces of vesicular myelin are often seen as debris within macrophages. Some have noted a close correlation of this change with the postmortem state or have found similar alterations in control autopsy nerves. These workers consequently dismiss this change as an artifact of delayed fixation (Honavar et al. 1991). Indeed, in GBS there is a tendency for this finding to be reported on autopsy studies. However, vesicular demyelination has also been observed in nerve biopsy specimens (Brechenmacher et al. 1987; Vital et al. 1985; Prineas 1972; Sumi et al. 1983). Although our experience confirms that this alteration is most often an artifact of delayed fixation or accompanying crush artifact, we have observed a number of examples in well-preserved nerve biopsy material, usually in diabetic neuropathy. Injecting sera from GBS patients into nerve culture or animal peripheral nerve produces vesicular demyelination (Hirano et al. 1971; Birchem et al. 1987; Saida et al. 1982; Brown et al. 1987). In some experimental allergic neuritis (EAN) models, vesicular demyelination takes place in close association with macrophage invasion (Dal Canto et al. 1975) or prior to the appearance of any inflammatory cells (Rosen et al. 1990). The latter authors offered the hypothesis that vesicular myelin degeneration is produced by humoral factors, most likely antibodies to myelin antigens, whereas cell-mediated immunity was involved in macrophage-mediated myelin stripping (Rosen et al. 1990). Vesicular demyelination has also been observed in experimental situations where immune attack does not operate, such as lead (Lampert and Schochet 1968) or tellurium (Lampert and Garrett 1971) toxicity and radiation injury (Masurovsky et al. 1967). Incubating peripheral nerve with calcium and a calcium ionophore results in the rapid occurrence of severe diffuse vesicular demyelination without axonal damage (Smith and Hall 1988), suggesting that vesicular myelin disintegration is a nonspecific consequence of any process that causes calcium leakage into the Schwann cell, with subsequent activation of endogenous phospholipase A2 (Smith and Hall 1988).
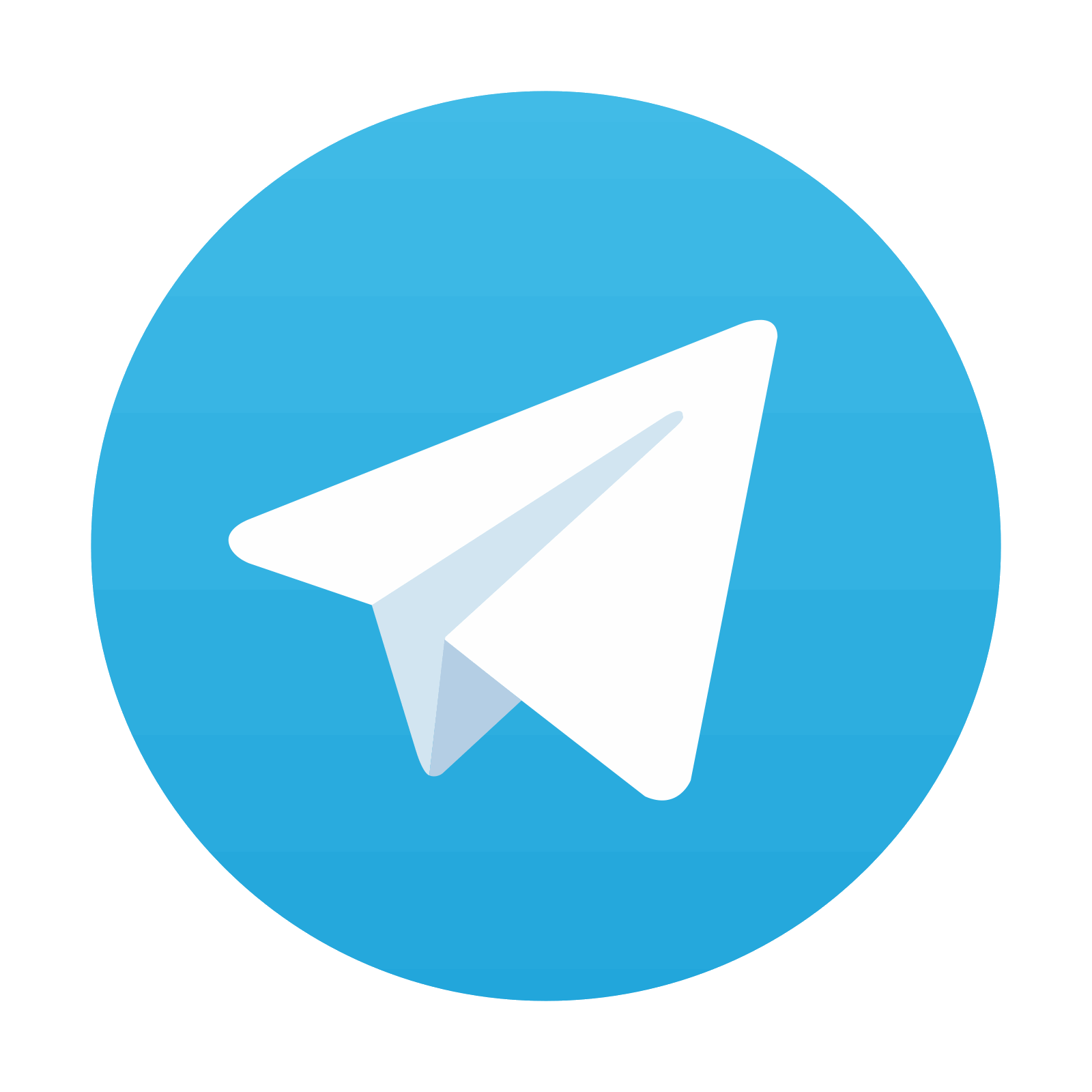
Stay updated, free articles. Join our Telegram channel
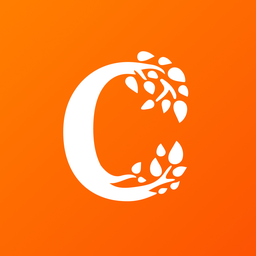
Full access? Get Clinical Tree
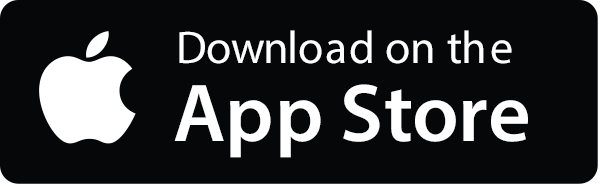
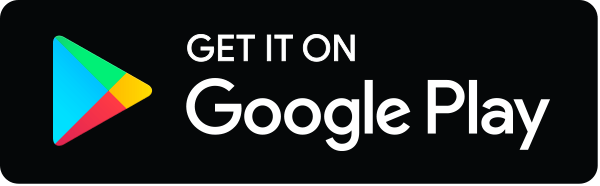
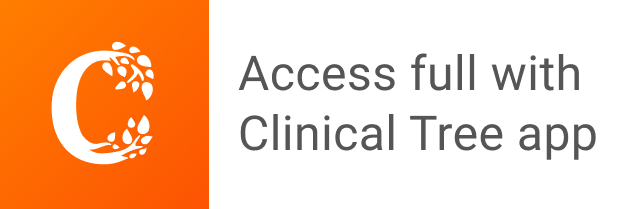