(1)
Facultad de Ciencias Médicas, Pontificia Universidad Católica Argentina, Buenos Aires, Capital Federal, Argentina
Abstract
The sympathetic and parasympathetic divisions of the ANS are integrated and regulated by a hierarchy of central structures. This central autonomic neural network has been identified and mapped in recent years and functional neuroimaging data largely support it. Such a network involves reciprocal connections, both direct and indirect, between the two efferent systems and with more cranial neural clusters located in the brainstem, hypothalamus, limbic system, and the insular cortex. As examples of that organization, this Chapter discusses the neural mechanisms that control arterial blood pressure, heart rate, breathing, immunity, and gastrointestinal function and how they vary in the three body configurations (wakefulness, NREM sleep, REM sleep).
Keywords
BrainstemCardiorespiratory homeostasisCarotid bodyCerebellumGastrointestinal functionImmunityMigrating motor complexNeural regulation of cardiovascular functionNeural regulatory system of breathingNucleus tractus solitariumParabrachial nucleusReticular formationObjectives
After studying this chapter, you should be able to:
Describe the location of forebrain and brainstem neurons that are components of central autonomic pathways.
Describe the neural mechanisms that control arterial BP and heart rate, including the receptors, afferent and efferent pathways, central integrating pathways, and the effector mechanisms involved, and how they vary in the three body configurations (wakefulness, NREM sleep, REM sleep) during a 24-h cycle.
Identify the location and functions of the dorsal and ventral groups of respiratory neurons, the pneumotaxic center, and the apneustic center in the brainstem and how they vary in the three body configurations (wakefulness, NREM sleep, REM sleep) during a 24-h cycle.
Describe the participation of the cerebellum in the autonomic posture.
Identify the roles and mechanisms of innate, acquired, humoral, and cellular immunity and how they vary in the three body configurations (wakefulness, NREM sleep, REM sleep) during a 24-h cycle.
Describe the functional significance of the gastrointestinal system, and its roles in nutrient assimilation, excretion, and immunity and how they vary in the three body configurations (wakefulness, NREM sleep, REM sleep) during a 24-h cycle.
At the Brainstem, Various Complex Autonomic Responses Are Coordinated
The sympathetic and parasympathetic divisions of the ANS are integrated and regulated by the hierarchy of central structures plotted in Fig. 1.2. This central autonomic neural network has been clearly identified and mapped in recent years and functional neuroimaging data largely support it. Such a network involves reciprocal connections, both direct and indirect, between the two efferent systems and with more cranial neural clusters located in the brainstem, hypothalamus, limbic system, and brain (e.g. insular cortex).
The brainstem is the region between the spinal cord and the diencephalon. It comprises the medulla oblongata, the pons, and the midbrain. Many sensory and motor nuclei and the different components of reticular formation are in the brainstem and lesions affecting this area have profound motor, sensory, and consciousness-related consequences, which is why a discussion of its organization is necessary for an understanding of the physiology of the ANS.
The brainstem is a site of somatic and autonomic motoneurons and different types of sensory neurons that are organized into anatomically identifiable columns corresponding to the cranial nerves [1]. The cranial nerves have three defined functions: (a) to provide somatosensory and motor innervation to the neck and head; (b) to provide innervation to the sense organs; (c) to provide parasympathetic preganglionic innervation to autonomic ganglia that control visceral function.
As in the spinal cord, somatic motor efferent neurons, autonomic efferent neurons (preganglionic) and second-order sensory afferent neurons coexist in the brainstem. Second-order sensory neurons receive afferent fibers from somatic or visceral primary sensory neurons located in ganglia outside the brainstem or in the sense organs (the only exception to this rule is the mesencephalic nucleus of the V pair, which contains primary sensory neurons).
What is particular in the brainstem is that, in the case of motor and sensory, somatic and visceral neurons of the cranial nerves are subdivided into anatomically segregated functional groups. This subdivision consists of two organizational principles:
- 1.
There are three types of motor neurons in the brainstem: somatic motoneurons, special visceral motoneurons, and general visceral motoneurons. Somatic motoneurons innervate the muscles of the face and neck derived from the myotome, with the same origin as the rest of the skeletal musculature. They send their fibers through the III, IV, VI, and XII cranial nerve pairs (voluntary ocular musculature and tongue). Special visceral motoneurons also innervate the striated musculature of the face and neck, but in this case, the muscles are derived from the gill arches (mastication, facial expression, larynx, pharynx). They send their fibers through V, VII, IX, X, and XI cranial nerve pairs. General visceral motoneurons provide parasympathetic preganglionic autonomic innervation. They send their fibers through the III, VII, IX and X cranial nerve pairs.
- 2.
There are four types of second-order sensory neurons in the brainstem: general somatic sensitivity, special somatic sensitivity, general visceral sensitivity, and special visceral sensitivity respectively. General somatic sensitivity comprises the sensitivity of face skin and oral and pharyngeal mucous membranes, and proprioception (V, VII, IX and X cranial nerve pairs). The special somatic sensitivity comprises that of the inner ear (VIII cranial nerve pair). The general visceral sensitivity comprises that coming from thoracic and abdominal organs (IX and X cranial nerve pairs). The special visceral sensitivity originates in the gustatory corpuscles (VII, IX, X cranial nerve pairs).
These seven functionally differentiable neuronal groups are grouped in columns along the brainstem, as represented in Fig. 4.1:

The somatic motor column , which contains motor neurons that innervate the extraocular and tongue muscles (in rostrocaudal order).
The special visceral motor column containing motor neurons that supply the muscles of the larynx, pharynx, face, and jaw. They are, in descending order: a) motor nucleus of the V cranial nerve pair (mastication); (b) motor nucleus of the VII cranial nerve pair (facial expression); (c) ambiguous nucleus (IX, X cranial nerve pairs; speech, swallowing); (d) nucleus of the spinal accessory nerve (XI cranial nerve pair).
The general visceral motor column , which contains the neuronal bodies of parasympathetic preganglionic neurons: (a) Edinger–Westphal nucleus (III), preganglionic to ciliary ganglion; (b) upper salivary nucleus (VII), preganglionic to the sphenopalatine ganglion (lacrimal gland) and submaxillary ganglion (sublingual and submaxillary glands); (c) inferior salivary nucleus (IX), preganglionic to the otic ganglion (parotid gland); (d) dorsal motor nucleus of the X pair: parasympathetic preganglionic to the thoracic and abdominal organs.
The general visceral afferent columns and the special visceral afferent columns form the nucleus tractus solitarium (NTS) , which comprises two parts: (a) rostral , which is the relay site for taste and general visceral afferents from the digestive tract; (b) caudal , which receives the afferents of the carotid body and the general visceral afferent from the bronchi and lungs. The perikarya of these primary sensory neurons are in ganglia associated with the VII, IX and X cranial nerve pairs, and their central extensions form the solitary tract, ending in the secondary neurons that form the solitary nucleus. From here, the portion that mediates sensitivity projects to the thalamus, whereas that corresponding to cardiorespiratory regulation establishes direct contact with the reticular and indirect formation with the limbic system (through the parabrachial nucleus, PBN).
The special somatic afferent column contains the vestibular and cochlear secondary relay neurons (VIII cranial nerve pair).
The general somatic afferent column contains secondary neurons of oral and oropharyngeal somatic sensitivities. It consists of three divisions separated rostrocaudally into: (a) the mesencephalic nucleus of the V cranial nerve pair (proprioception of facial and mandibular muscles, the only case of primary sensory neurons in a brainstem) receives afferents from mucous and muscles; (b) the main nucleus of the V cranial nerve pair receives afferents from mucous and muscles; (c) the spinal nucleus of the V cranial nerve pair.

Fig. 4.1
Nuclei of cranial nerves and column organization of the brainstem. Modified with permission from Cardinali [3]
In summary, there are three basic principles in the organization of the cranial nerves:
- 1.
Most motor nuclei are linked to individual cranial nerves.
- 2.
Relay nuclei in the sensory pathways receive fibers from various cranial nerve pairs, e.g., the NTS receives taste information brought by the VII, IX, and X pairs, following the general principle that somatotopic sensory location ends in the same central area, regardless of the route it follows.
- 3.
Neurons with similar functional properties occupy the same positions in the brainstem.
The NTS , located in the dorsal part of the medulla oblongata, and secondarily the NPB, constitute the main centers of central relay of visceral sensory information (Fig. 4.2). The main afferent inputs of the NTS comprise fibers from the cardiovascular, respiratory, gastrointestinal, and neuroendocrine–immune systems, along with gustatory collaterals and somatoesthetic sensitivity.


Fig. 4.2
Visceral sensory input to the nucleus tractus solitarius (NTS) brain relevant to the control of food intake and energy balance. All along the alimentary canal, various mechano- and chemosensors are located that transmit food- and nutrition-related signals via primary visceral afferents in the trigeminal (V), facial (VII), glossopharyngeal (IX), and vagus nerve (X) to the brainstem
The NTS is the primary integrative center for cardiovascular control and other autonomic functions in the CNS. The NTS has long been identified as a site where the first synapse of the baroreceptor reflex is located. Therefore, the NTS, in addition to other key central nuclei in the hypothalamus and other forebrain regions, play important roles in mediating cardiovascular responses to acute stresses. The NTS not only integrates convergent information, but itself is the site of substantial modulation. Evidence demonstrated several neurotransmitters or neuromodulators such as Glu, NE, E, ACh, 5-HT, NO, angiotensin II, arginine vasopressin (AVP), β-endorphin, enkephalins, NPY, adenosine and insulin [2].
By means of neural circuitry mapping techniques a viscerotopic map was traced in the NTS and in the neuronal groups of the dorsal nucleus of the X cranial nerve pair. These data support the notion that vagal information in the gut–brain axis maintain specific pathways from the gastrointestinal tract through individualized vagal branches. The viscerotopic organization of the gut–brain afferent loop correlates with a parallel map in the efferent limb of the brain–gut axis forming a network ideally suited to mediate cephalic, gastric, hepatic, and intestinal reflexes accompanying the food intake.
The NTS is subdivided into several subnuclei, which play different functional roles according to the visceral afferents they receive (Fig. 4.2). Thus, cardiovascular afferences terminate predominantly in the dorsal subnucleus, pulmonary afferents in the ventral subnucleus, and gustatory input in the parvicellular subnucleus, whereas the caudal commissural subnucleus receives afferences from all visceral components. Projections from the NTS transmit a wide range of visceral information to higher nuclei, and establish circuits of reciprocal connection with regions of the reticular formation (particularly PBN and periaqueductal gray matter), hypothalamus, amygdala, limbic system, and insular cortex.
The descending efferent pathways of these circuits innervate, directly or through synapses in the NTS, the parasympathetic preganglionic neurons and the sympathetic intermediolateral columns. The descending autonomic pathways mainly travel ipsilaterally in the anterior portion of the lateral cord. Using neuroimaging (functional magnetic resonance imaging, fMRI; positron emission tomography, PET) the asymmetry and lateralization of the ANS function was verified.
The PBN plays a key role in the central autonomic network, as an intermediate between the brainstem reflex control and behavioral control systems at the diencephalic and telencephalic levels (Fig. 4.2). The most relevant parts of the PBN for controlling food intake and energy homeostasis are the medial and lateral subdivisions. The neurons located in the upper lateral nucleus of the lateral subdivision of PBN are particularly important for the control of food intake, and send dense projections to the ventromedial nucleus (VMN) of the hypothalamus related to the control of satiety. The upper lateral nucleus of the PBN has a large concentration of CCK-expressing neurons that are related to the induction of satiety and are activated by circulating leptin.
The central projections on the preganglionic autonomic neurons come mainly from the hypothalamus and various brainstem nuclei (dorsal raphe nucleus, DRN, locus coeruleus, LC, ventrolateral medulla reticular formation). The role of these central pathways is to maintain a tonic state of excitability of preganglionic autonomic neurons, modulate segmental reflexes, and generate organized patterns of activity in different functional groups of preganglionic neurons.
In the viscera, there are numerous receptors, such as osmoreceptors, baroreceptors, glucoreceptors, etc., which respond to changes in the internal environment. Their afferents participate in different autonomic reflexes of homeostatic importance. These receptors are the basis of interoception, and presumably they can be the physical substrate of what from a psychological point of view is called the preconscious.
A key question is whether nuclei such as the NTS or PBN are predominantly relay nuclei, or do they transform incoming visceral information? It is noteworthy that contrary to popular anatomical terminology, there is no need for relay nuclei because axon potentials do not need to be boosted by the synapse. A chemical synapse on its own simply adds an unavoidable delay.
Neurons form synapses in a nucleus to transform or change the incoming signal. The information obtained in the nuclei of the dorsal column (Goll and Burdach nuclei) in the somatosensory system indicates the existence of several transformations. On the one hand, there is differential convergence that results in a distorted viscerotopic representation of the body. In addition, because of the lateral inhibition, the discrimination of the stimuli is accentuated. As in other sensory territories, stimulus in the center activates NTS neurons whereas stimulus in the surround, through inhibitory feedback, inhibits the same NTS neurons. Another function is cortical gating: corollary discharge selectively gates input based on motor output [3].
The existence of the gating control exerted by superior mesencephalic, diencephalic, and cortical structures on the afferent information that arrives from receptors is exemplified by pain. The main function of this input control (central analgesia) is to suppress the irrelevant information originated in the periphery, allowing only that of sufficient intensity to have an adaptive meaning to pass. As seen in Figs. 4.3 and 4.4, the convergence of visceral and cutaneous afferents on the same second-order neural groups results in visceral pain being experienced on a portion of the cutaneous surface (referred pain). This referred pain is verified in the portion of the cutaneous surface of embryological origin similar to the affected viscera (dermatome) [4].



Fig. 4.3
The main function of this afferent control (central analgesia) is to suppress the irrelevant information originating at the periphery, only allowing that of sufficient intensity to have an adaptive meaning to pass. Modified with permission from Cardinali [3]

Fig. 4.4
The convergence of visceral and cutaneous afferents on the same second-order neural groups results in visceral pain being experienced on a portion of the cutaneous surface (referred pain). Modified with permission from Cardinali [3]
Monoaminergic Systems in the Brainstem Modulate 24-h Rhythms in Physiological Function
The reticular formation is composed of neurons that do not correspond to the different functional columns of the brainstem mentioned above. It is convenient from an anatomical point of view to analyze the reticular formation in the medial–lateral sense, thus distinguishing (a) the raphe nuclei at both sides of the medial line; (b) a magnocellular region; (c) a parvicellular region.
Pons reticular formation and midbrain reticular formation have different functions. Most neurons in the reticular formation display a great diversity of connections, diffusely distributed, both with upper centers and toward the spinal cord (distribution “in a spider web,” Fig. 4.5). Based on the neurotransmitter employed, the following neural groups are distinguished, most of them with locations in the brainstem:




- 1.
Noradrenergic system : located in the LC and contiguous nuclei, it provides innervation to the entire brain, including the spinal cord (Fig. 4.6). Except for a small intrahypothalamic group of neurons, this is the only source of central noradrenergic innervation. As discussed in Chap. 2, its role is fundamental in the maintenance of wakefulness and its alteration is linked to emotional illnesses such as depression, their spinal projection participating in muscular tone control and gait generation. The different NE receptors have been analyzed in Chap. 3.
- 2.
Dopaminergic system : several cell groups are in the midbrain (e.g., the substantia nigra pars compacta and the VTA, Fig. 4.7). They provide dopaminergic innervation to the basal ganglia, hypothalamus, limbic system, and neocortex. Four major dopaminergic systems are found in the CNS: (a) nigrostriatal; (b) mesolimbic; (c) hypothalamic tuberoinfundibular; (d) retinal. The nigrostriatal projection (neuronal bodies in the substantia nigra pars compacta) participates in the regulation of function of the basal ganglia, whereas the mesolimbic projection (neuronal bodies in the VTA, or A10) is involved in emotional states, psychiatric diseases, and drug addiction. The tuberoinfundibular system (neuronal bodies in the arcuate nucleus, ARC, of the hypothalamus), participates in the control of prolactin (PRL) secretion . The retinal dopaminergic neurons are a subgroup of amacrine interneurons. Six major types of dopaminergic receptors have been identified and their different isoforms have been cloned. These types are called D1, D2a, D2b, D3, D4, and D5. They all have the seven-peptide hydrophobic sequences that indicate their association with G proteins. Functionally, they can be differentiated by their nature into (a) excitatory (class D1, comprising D1 and D5) associated with a stimulating G protein and with the activation of an adenylate cyclase; (b) inhibitory (class D2, comprising D2, D3, and D4) associated with an inhibitory G protein and inhibition of an adenylate cyclase.
- 3.
Serotoninergic system : comprising the DRN (a continuation of the periaqueductal gray matter; Fig. 4.8). DRN are the origin of almost all serotonergic innervation of the brain. The serotonergic receptors (5-HT-1, 5-HT-2, 5-HT-3, 5-HT-4, 5-HT-5, 5-HT-6, and 5-HT-7) are classified by the gene superfamily corresponding to:
- (a)
The superfamily of receptors associated with G protein, including 5-HT1A, 5-HT1D, 5-HT1E, and 5-HT1F (all inhibit adenylate cyclase), the 5-HT2 receptor subfamily (5-HT2A, 5-HT2B, and 5-HT2C subtypes, all stimulate the synthesis of phosphoinositides and phospholipase C), and 5-HT-4, 5-HT-5, 5-HT-6, and 5HT-7 receptors (which stimulate adenylate cyclase). These receptors, especially 5-HT-1 (5-HT1D), are linked to the normal and pathological regulation of cerebral flow. 5-HT1A agonists have anxiolytic action
- (b)
The superfamily of receptors that directly control channels, corresponding to the 5-HT 3 receptor.
- (a)
- 4.
Cholinergic system : there is a dense cholinergic innervation of the thalamus, striatum, limbic structures, and cerebral cortex (Fig. 4.9). Except for the striatum , which is mainly intrinsic (from local interneurons), in most other regions cholinergic innervation is extrinsic. The medial–septal area (with the diagonal band of Broca) is the origin of cholinergic innervation of the hippocampus (septohippocampal neurons). The nucleus basalis of Meynert is the origin of the cholinergic innervation of the neocortex and amygdala (Fig. 4.9). The group of cholinergic neurons of the pontomesencephalic reticular formation (PPT/LDT) are the source of cholinergic thalamic innervation (Fig. 4.9). The neurons of these nuclei participate in the waking reaction, and in the onset of REM sleep (they activate REM-on cells, Chap. 2). The alteration of the aforementioned cholinergic systems coexists with the alterations of the memory and learning, such as those observed in Alzheimer’s disease (AD).
- 5.
Histaminergic system : although it has been linked to this system with monoaminergic neurons “in a spider web,” its location is not mesencephalic, but diencephalic, in the tuberomammillary nucleus (TMN) of the posterior hypothalamus (Fig. 4.10). Its receptors are a class of G protein-coupled receptors comprising four groups: H1 (associated with the circadian cycle, itching, systemic vasodilatation, and bronchoconstriction; H2 (tachycardia, stimulation of gastric acid secretion, smooth muscle relaxation, inhibition of antibody synthesis, T cell proliferation, and cytokine production); H3 (presynaptic autoreceptor, decreasing ACh, 5-HT and NE in CNS); H4 (which mediates mast cell chemotaxis).

Fig. 4.6
Principal noradrenergic projections of the reticular formation . Modified with permission from Cardinali [3]

Fig. 4.7
Principal dopaminergic projections of the reticular formation . Modified with permission from Cardinali [3]

Fig. 4.8
Principal serotonergic projections of the reticular formation . Modified with permission from Cardinali [3]

Fig. 4.10
Histaminergic projections originating in the tuberomammillary nucleus (TMN) . (a) Distribution in a sagittal view. (b) Hypothalamic location of the TMN. Modified with permission from Cardinali [3]
As already mentioned, in most cases, these systems do not participate in point-to-point communication, but rather their diffuse distribution points to a general permissive role for other brain processes. This anatomical arrangement offers an ideal anatomical substrate for diffuse functions of alertness, emotionality, and neurovegetative control. In addition to the role discussed in Chap. 2 in the regulation of sleep/wake rhythm, the functions of reticular formation include:
Activation of the CNS to trigger specific behaviors and control of alertness.
Modulation of medullary reflexes and muscle tone, through the reticulospinal tracts.
Modulation of locomotion (descending pathways of the LC).
Modulation of the ventromedial and dorsolateral spinal motor neurons (LC, DRN).
Participation in respiratory and cardiovascular control.
Participation in the control of different visceral responses, such as urination, vomiting or defecation.
Modulation of the flow of nociceptive information in the posterior horn of the medulla, through reticulospinal projections, mainly serotonergic.
Rather than an apparent reticular structure with little order, the monoaminergic areas of the brainstem represent a modular somatotopic organization like other brain areas, such as the neocortex. For example, the periaqueductal serotonergic DRN neurons have columns composed of afferent, output neurons and interneuronal circuits organized viscerotopically. Thus, important functions linked to these areas, such as defensive reactions, analgesia, and autonomic regulation, are integrated in overlapping longitudinal columns, and different types of aversive or painful stimuli trigger specific somatic, vegetative, and nociceptive programs.
24-h Rhythms in Cardiovascular Control
A schematic representation of the extrinsic and intrinsic innervation of the heart is depicted in Fig. 4.11. The neural regulation of the cardiovascular system , controlled by arterial mechanoreceptors, operates as a reflex arc (Fig. 4.12). Elevations or falls in BP cause a proportionally greater or lesser deformation of the arterial walls, which are encoded by baroceptors to a greater or lesser frequency of action potential firing. This information is carried to the CNS by afferent nerve fibers: (a) the aortic depressor nerve , which travels along the X cranial nerve pair; (b) the sinus nerve (Hering’s nerve), which travels along the IX cranial nerve pair [5].



Fig. 4.11
Schematic representation of the extrinsic and intrinsic innervation of the heart

Fig. 4.12
Schematic representation of neurohumoral responses triggered by the stimulation of vascular mechanoreceptors by changes in blood pressure (BP) at the three body configurations in a 24-h cycle. ADN aortic depressor nerve, HN Hering’s nerve, rVLM rostral ventrolateral medulla, cVLM caudal ventrolateral medulla, AN ambiguus nucleus. DMN dorsomedial nucleus of vagus, SNS sympathetic nervous system, ANP atrial natriuretic peptide, HR heart rate, SV systolic volume, VC venous capacitance, TPR total peripheral resistance, CO cardiac output, VR venous return. The figure was prepared in part using image vectors from Servier Medical Art (www.servier.com), licensed under the Creative Commons Attribution 3.0 Unported License (http://creativecommons.org/license/by/3.0/)
Primary afferent axons from the baroreceptors project to the caudal region of the NTS, where they synapse onto second-order neurons , which in turn send excitatory glutamatergic projections onto inhibitory neurons within the region of the caudal ventrolateral medulla. These inhibitory neurons synapse directly onto excitatory neurons within the rostral ventrolateral medulla and serve to inhibit the spontaneous activity of premotor sympathetic neurons (Fig. 4.12). Glutamatergic neurons from the NTS also project to the X cranial nerve pair nuclei that in turn project to the heart via the X cranial nerve pair. The vagal output originates from preganglionic neurons located in the dorsal motor nucleus of the vagus and in the ventrolateral portion of the ambiguous nucleus (AN) in the medulla.
Studies in awake human subjects, in which fMRI was coupled with microelectrode recordings of muscle sympathetic nerve activity, has confirmed the operation of these medullary nuclei in humans [6]. Specifically, information on changes in the rostral ventrolateral medulla indicated that it is implicated in elevated sympathetic outflow associated with several cardiovascular diseases including hypertension and heart failure related to physical inactivity [7].
In the heart, the vagal postganglionic fibers cause bradycardia, when the initial stimulus is an increase in BP, and tachycardia, when the stimulus is a decrease in BP (Fig. 4.12). Other glutamatergic neurons in the NTS project to the caudal ventrolateral medulla, exciting it or not, depending on the increase or decrease in BP. GABAergic neurons at the caudal ventrolateral medulla project to the rostral ventrolateral area, inhibiting it (if the initial stimulus is a BP elevation) or not (when BP decreases). The rostral ventrolateral area is the site of sympathetic premotor neurons that project to the intermediolateral column of the spinal cord, the site of preganglionic neurons to the cardiac intrinsic and extrinsic ganglia (Fig. 4.11), and to the sympathetic peripheral ganglia innervating resistance and capacitance vessels. This sympathetic activity is thus inhibited during increases in BP or not inhibited during transitional decreases in BP [8].
Thus, when the triggering reflex stimulus is an increase in BP, there is, reflexively, a vagal activation and inhibition of the sympathetic output, with a consequent reduction in heart rate, systolic volume, and total peripheral resistance, and an increase in venous capacitance. This decreases the venous return to the heart, determining a decrease in BP and its return to baseline (Fig. 4.12). On the other hand, when the triggering stimulus is a decrease in BP, the vagus nerve is not activated and the sympathetic input is not inhibited. This causes an increase in heart rate, systolic volume, and total peripheral resistance, and a decrease in venous capacitance. Consequently, there is an increase in the venous return, which helps to increase cardiac output further (Fig. 4.12). These responses, triggered neurally, are extremely fast, correcting, in seconds, BP swings up or down the control values.
Orthostatic pooling of blood begins almost immediately upon the change from the supine to the upright posture. The main sensory receptors involved in orthostatic cardiovascular reflex adjustment are the arterial baroreceptors located in the carotid sinuses and aortic arch and mechanoreceptors located in the heart and lungs [9]. A decrease in BP, as occurs on assumption of the upright posture, removes this tonic inhibition with a resultant decrease in vagal outflow and an increase in sympathetic activity causing an increase in heart rate, cardiac contractility and vasomotor tone. Central modulation of vasomotor outflow is reinforced by local vasoconstrictor mechanisms, such as the veno-arteriolar axon reflex and a myogenic response. The veno-arteriolar axon reflex is triggered when venous pressure exceeds 25 mmHg, which results in vasoconstriction of the corresponding arteriole and is reported to elicit up to 30–45% of the total vasoconstriction in the legs in the upright posture [9].
The sympathetic and parasympathetic components of the ANS play a crucial role in maintaining cardiovascular homeostasis and enabling the body to respond to physiological stressors. Neurogenic mechanisms are not only essential for maintaining and regulating arterial BP, but also play a crucial role in regulating the distribution of blood flow between and within vascular beds. The sympathetic component of the ANS plays the predominant role in regulating vascular tone and whole-body hemodynamics via its effects on both resistance and capacitance vessels. By contrast, the overall contribution of the parasympathetic nervous system to the regulation of vascular tone and hemodynamics is small compared with its primary regulatory role in mediating negative chronotropic and inotropic effects on the heart [10].
The vascular endothelium plays an essential role in the regulation of blood vessel tone and cellular activity, helping to maintain a healthy vessel [11]. Endothelial cells produce several important vasoactive substances including NO, prostacyclin, endothelium-derived hyperpolarizing factor, endothelin, vasoactive prostanoids, and ROS. These factors, and other endothelium-derived substances, also modulate local thrombotic and inflammatory pathways influencing the progression of atherosclerosis and its complications. Exposure to risk factors for atherosclerosis and the presence of circulatory diseases, including atherosclerosis and heart failure, leads to endothelial activation, associated with reduced NO bioavailability and expression of proinflammatory cytokines, chemokines, selectins, and adhesion molecules. This enhances vasoconstrictor tone and promotes a proatherogenic milieu.
Several hormonal mechanisms are also involved in BP control, including the release of catecholamines, angiotensin II, aldosterone, AVP, oxytocin, and atrial natriuretic peptide (ANP), which act by supporting the maintenance of baseline BP, intensifying and prolonging the cardiovascular responses for minutes or even hours, making BP control more effective, especially in situations of prolonged elevations or falls of BP (hemorrhage, dehydration, drug reactions, etc.; Fig. 4.12). The actions of signaling molecules that are not classically viewed as such, e.g., cytokines and ROS, must also be considered [12].
Circadian clock genes are expressed in the heart and aorta and these genes and approximately 4–6% of the cardiac protein genes showed circadian rhythms in transcription [13]. Ex vivo experiments demonstrate that varied functions of the heart and aorta are dependent on the time in which tissues are collected. In murine knock-out models of circadian genes, suppression of Bmal1 in cardiomyocytes results in an abnormal electrocardiogram (ECG) with RR and prolonged QRS intervals. The hearts of Bmal1 knockout mice were more susceptible to arrhythmia. Other studies have revealed that removal of Bmal1 in endothelial cells or vascular smooth muscle cells alters the diurnal variation of BP. These findings are consistent with the presence and importance of circadian genes in the cardiovascular system [13, 14].
The alteration of normal day–night cycles, such as jet lag or shift work, leads to the desynchronization between the central and peripheral clocks and the deregulation of the clock genes (Chap. 8). Restoration of a normal daytime rhythm rescues from these changes, suggesting that maintaining a normal rhythm is crucial for cardiovascular health.
Cardiovascular function changes significantly in the three body configurations during the 24-h cycle [15]. BP decreases during NREM sleep and becomes variable in REM sleep . During REM sleep, transient BP increases of up to 40 mmHg occur that coincide with the phasic events of this stage of sleep in conjunction with vasoconstriction in the skeletal muscles. Pulmonary artery pressure remains stable. Variation of sleep-related BP can be described by a square wave function with changes in the onset and end of sleep and relatively constant values during sleep. This fall in BP during sleep is important for cardiovascular health [15].
The values of systolic pressure drop 15 mmHg or more during sleep and are heavily influenced by the S Process described in Chap. 2. That is, the BP drop accompanies the presence of sleep, regardless of the time of day at which it occurs. An initial fall in BP due to postural change and darkness (~ 7 mmHg) is followed by a period of instability when sleep is unstable (stage N1 of sleep) and by an abrupt drop once stable sleep is achieved (N2–N3 stages of sleep; ~ 7 mmHg). Within each sleep phase the BP is constant, in the NREM sleep , the values are lower than in wakefulness, and in REM sleep they are similar to those of relaxed wakefulness. Although there are transient increases in association with phasic events of REM sleep , the major disturbance of BP during sleep is in the awakening. BP at the end of sleep shows a rise largely due to postural changes. The magnitude of the changes is greater when awakening occurs at the N2 sleep stage compared with awakening in the REM stage [15].
In the case of the heart rate the closest approximation is to a sinusoidal pattern related to the central temperature and compatible with the influence of the C process (circadian), i.e., the changes occur regardless of sleep. The lowest point of the oscillation is in the middle of the night and remains, albeit attenuated, in individuals who are deprived of sleep. As for BP, at the beginning of sleep, there is a fall in the heart rate with two components (preparation for sleep; when sleep becomes stable). There is a close relationship between heart rate and metabolic heat production because of its circadian dependence. Heart rate is higher during the REM sleep phase, with transient tachycardia in relation to REM phasic events (Fig. 4.13).


Fig. 4.13
Modulation of cardiac activity at the three body configurations in a 24-h cycle. Letters designate reflex loops (a: baroreflex; b: chemoreflex; c: respiration). The brain stem centers (BS) and central autonomic network including midcingulate cortex (MCC), insula (INS), amygdala (AMYG) are depicted. Relative changes in the heart rate (HR), sympathetic nervous system. In red increases and in blue decreases in activity are shown. Redrawn from Chouchou and Desseilles [16]. The figure was prepared in part using image vectors from Servier Medical Art (www.servier.com), licensed under the Creative Commons Attribution 3.0 Unported License (http://creativecommons.org/license/by/3.0/)
Heart rate variability (HRV) analysis combined with brain imaging has identified a close connectivity between autonomic cardiac modulation and activity in brain areas such as the amygdala and insular cortex during REM sleep, but no connectivity between the brain and cardiac activity during NREM sleep [16]. There is also evidence for an association between HRV and the intensity and emotionality of dreams.
Brief awakenings are a characteristic of normal sleep. They occur with high frequency (more than 15–20 per night) and are a normal situation in which an increase in heart rate (≥8 beats per minute), BP (<15 mmHg) and peripheral vasoconstriction are observed. Awakenings occur in both NREM sleep and REM sleep . There are two components of awakenings. The first component is a transient peak of heart rate and BP that occurs within 3–6 s of the event. The second component is dependent on the previous wake period. Most awakenings are brief and there is no such second component. For many normal individuals, the cardiovascular activation response occurs at a level of excitation below the occurrence of α changes in the EEG (cortical awakening). There are large individual differences in cardiovascular response to awakenings and interindividual variability of systolic BP response in an awakening can reach about 15 mmHg. Healthy individuals with large activating cardiovascular responses are at increased risk for cardiovascular disease because of frequent awakenings [17].
In NREM sleep , there is a period of relative autonomic stability with vagal predominance and increased gain of baroreceptors. A sinusoidal modulation of heart rate exists because of the coupling of cardiovascular and respiratory regulation centers, resulting in respiratory sinus arrhythmia. During inspiration, the frequency briefly accelerates to accommodate the increased venous return, resulting in increased cardiac output, whereas during expiration, a progressive decrease in heart rate occurs. This normal sinus heart rate variability, particularly during NREM sleep , is generally indicative of a state of heart health; thus, the absence of sinus arrhythmia has been associated with heart disease and advanced age [18].
Cardiac- and respiratory-related rhythms are observed in nerve activity supplying the heart and blood vessels [19]. These rhythms arise from phasic inputs related to cardiac/pulse or ventilation-related afferent activity and/or a common cardiorespiratory CNS network. Much of this heart rate fluctuation is linked to the phase of respiration (i.e., respiratory sinus arrhythmia) and its loss is a prognostic indicator of morbidity and mortality. Another possible origin of sympathetic rhythms is independent CNS oscillators. Separate oscillators, which are able to couple, may drive activity to different sympathetic nerves, and sympathetic neurons regulating the same target may be influenced by populations of weakly coupled or uncoupled oscillators [19]. Two major hypotheses have been proposed to account for cardiac- and respiratory-related rhythms in sympathetic discharges. The classic view holds that these rhythms are imposed upon sympathetic discharge by “external” inputs. The observation of a nonrespiratory and noncardiac-related sympathetic rhythm suggests that sympathetic rhythms might not arise exclusively from phasic inputs to tonic sympathetic tone generating networks [19]. Loss of vagal tone in cardiovascular diseases can be demonstrated by the diminished change in heart rate on administration of a vagolytic drug such as atropine and by the loss of respiratory sinus arrhythmia. The burst of cardiac vagal activity originates centrally at the level of the preganglionic neurons in the AN, which are inhibited during inspiration, but excited during postinspiration. The changes in BP during respiration are inversely related to the respiratory effort increases in BP, resulting in a decrease in respiratory rate. This effect is potentiated during NREM sleep in which small reductions in BP lead to increases in respiratory rate. These pauses and increases of respiratory rate serve as compensatory mechanisms to normalize BP and their disappearance in infants predisposes to a sudden death.
Sympathetic nerve activity is relatively stable during NREM sleep , and cardiovascular activity is reduced to more than half of that observed in the vigil at the N3 NREM sleep stage. NREM sleep, with relative hypotension, bradycardia, and reduced cardiac output and systemic vascular resistance, provides a “daily vacation” for heart activity. Bradycardia is mainly of a vagal origin, whereas hypotension is mainly attributable to a reduction in sympathetic vasomotor tone.
In the transition from NREM to REM sleep, abrupt vagal discharges can lead to pauses in cardiac rhythm or to asystolia. As discussed in Chap. 2, REM sleep occurs at 90-min intervals and several essential homeostatic mechanisms are disrupted during this period. The increase in limbic activity during REM sleep leads to significant sudden increases in cardiac sympathetic nerve activity at the level of the coronary vessels (Fig. 4.13). The baroreceptor gain is reduced and the heart rate fluctuates markedly, with marked episodes of tachycardia and bradycardia. Vagal tone is generally suppressed during REM sleep with very irregular breathing patterns that can lead to hypoxemia, particularly in patients with pulmonary or cardiac disease. Except for the diaphragm and cricopharyngeal sphincter there is hypotonia of the muscles of the airways. During sleep apnea , respiratory activity can be stopped for central or peripheral reasons several times each night, with adverse consequences for normal cardiorespiratory activity.
Changes in coronary blood flow occur at sleep states and during transitions between sleep states. In monkeys, nighttime coronary flow increases sporadically up to 100% during sleep. These periodic oscillations in blood flow are not associated with alterations in cardiac work or BP. Concomitant with sudden increases in heart rate during REM sleep, abrupt increases in coronary pressure and corresponding decreases in coronary vascular resistance were observed. These phenomena are seen predominantly during REM sleep in phase with POG events in EEG and ocular movements. There were no significant changes in mean BP. As the heart rate is elevated during sudden increases in coronary flow, increased cardiac metabolic activity was assumed to be the cause of coronary vasodilation. These sudden increases in coronary blood flow appear to be the result of increased adrenergic discharge as they were suppressed by removal of the corresponding sympathetic ganglia.
During severe stenosis of the coronary artery a decrease in coronary arterial blood flow is observed during REM sleep instead of the increases found in normal subjects. In these patients, there is a strong correlation between the magnitude of the increase in heart rate and the decrease in coronary flow. The link between REM sleep and the occurrence of myocardial ischemia in coronary patients is consistent.
24-h Rhythms in Respiratory Control
The spontaneous rhythm alternating between inspiration and expiration is produced by the automatic generation of a basal pattern in the brainstem. This spontaneous and stereotyped rhythm is modified by metabolic changes such as changes in pH or partial gas concentrations in the blood, or by mechanical changes (e.g., postural). Respiratory rate and tidal volume are modifiable to allow modifications of the internal environment. Interruptions of breathing (apnea) can be generated to allow phonation or swallowing.
The regulatory system of breathing includes: (a) a control center located in the CNS at the level of the brainstem, where the neural activity that triggers breathing starts; (b) effector muscles that produce ventilation (the respiratory muscles, especially the diaphragm); (c) a set of sensors located in the lungs and central and peripheral chemoreceptors that regulate respiratory activity [20].
The respiratory centers are divided into four major groups, two groups in the medulla and two in the pons (Fig. 4.14). The two groups in the medulla are the dorsal respiratory group and the ventral respiratory group. The two groups in the pons are the pneumotaxic center also known as the pontine respiratory group, and the apneustic center. The exact location of the apneustic center is not yet defined. The inspiratory center (dorsal respiratory group) is in the dorsal portion of medulla, in the NTS.


Fig. 4.14
The respiratory centers are divided into four major groups. The two groups in the medulla are the dorsal respiratory group (DRG) and the ventral respiratory group (VRG) . The two groups in the pons are the pneumotaxic center and the apneustic center (whose exact location is not yet defined). The inspiratory center (DRG) is in the dorsal portion of the medulla, in the NTS. The expiratory center (VRG) is in the ambiguous nucleus. For other details, see the text
The expiratory center (ventral respiratory group) is in the AN , in the anterolateral part of the medulla (Fig. 4.14). The AN consists of a rostrally to caudally extending column of neurons expressing respiratory-related activity, with subregions containing motoneurons that innervate the muscles of the larynx and pharynx that are not considered part of the ventral respiratory group. It generally causes expiration, but can cause either expiration or inspiration depending upon which neuron in the group is stimulated.
The expiratory center sends an inhibitory impulse to the apneustic center , which is presumably located in the lower part of the pons and which releases stimulatory impulses to the inspiratory center causing inspiration. It receives an inhibitory impulse from the pneumotaxic center and from stretch receptors of the lung via the X cranial nerve pair and discharges inhibitory impulse to the expiratory center. The pneumotaxic center, located in the upper part of the pons, in the PBN , controls both rate and pattern of breathing and limits inspiration (Fig. 4.14).
Both the dorsal respiratory group and the other sub-nuclei of the NTS are the primary sites for vagal afferent projection of the lungs and afferents and chemoreceptors of the carotid and aortic baroreceptors. The NTS, including the dorsal respiratory group, is a key site integrating sensory information from the lung, in addition to information on the levels of arterial PCO2, PO2, and pH.
The ventral respiratory group and dorsal respiratory group contain both bulbospinal respiratory premotoneurons (i.e., neurons that project to spinal motoneurons, which in turn innervate the respective respiratory pump and abdominal muscles of breathing), and propriobulbar neurons (i.e., neurons that project to, and influence the activity of, other medullary respiratory neurons, but do not themselves project to motoneurons). The hypoglossal, trigeminal, and facial motor nuclei also innervate muscles important to pharyngeal motor control and the maintenance of upper airway patency.
In mammals, the Bötzinger complex is a group of neurons located in the rostral ventrolateral medulla and ventral respiratory column. In the medulla, this group is located caudally to the facial nucleus and ventrally to the AN. The Bötzinger complex plays an important role in controlling breathing and responding to hypoxia. It consists primarily of glycinergic neurons, which inhibit respiratory activity.
The Bötzinger complex has projections to the NTS, phrenic pre-motor neurons in the medulla, phrenic motor neurons in the cervical spinal cord, the dorsal respiratory group, and the ventral respiratory group (Fig. 4.14). Bötzinger complex neurons are intrinsic pacemakers that are important to the generation of the basic respiratory rhythm and the expression of rhythmic neuronal activity elsewhere in the respiratory network. Respiratory rhythm-generating pre-Bötzinger complex neurons coexpress μ-opioid and neurokinin-1 receptors (i.e., the receptors for substance P) that slow and increase the respiratory rate respectively. The presence of μ-opioid receptors in pre-Bötzinger complex neurons explains the respiratory rate depression that follows the administration of opioid drugs. During inspiration, the central respiratory drive potential is transmitted to phrenic and intercostal motoneurons via monosynaptic connections from inspiratory premotor neurons of the dorsal respiratory group.
Central sensors that detect changes in CO2 are located on the ventral surface near the entrance of the VIII and XI cranial nerve pairs. These chemoreceptors respond to the local application of CO2 or acids and are inhibited by anesthetics and local cold. These chemoreceptors are not in direct contact with blood, but are bathed in CSF and respond to changes in both arterial PCO2 and CSF pH.
Carbon dioxide diffuses easily through the blood–brain barrier, but H+ does not. Thus, the stimulus produced by increased ventilation is the increase in PCO2, which, after crossing the blood–brain barrier, causes a fall in pH in brain tissue. Alterations of PaCO2 are rapidly transmitted to the CSF, which has little CO3H2 as a buffer. After an acute change in arterial PaCO2, there is an even greater change in PCO2 in the CSF. The response time constant is about 60 s.
Peripheral sensors are located in the carotid and aortic bodies [21]. They measure PO2, PCO2, and arterial pH. They are sensitive to the decrease in PaO2 that is measured directly and induces hyperventilation. Denervation of peripheral chemoreceptors leaves hypoxia without its fundamental homeostatic regulatory mechanism and because of hypoxia, CNS depression occurs. Increases in PaCO2 and the decrease in arterial blood pH stimulate these receptors less, but amplify their response to hypoxemia.
The carotid body , a highly vascular tissue, receives afferent innervation from the carotid sinus nerve, which is a branch of the glossopharyngeal nerve. The increased sensory activity of the carotid body is maintained during the entire period of hypoxia with little adaptation. Thus, the exquisite sensitivity and the rapid response to a wide range of hypoxic intensities with little or no adaptation make the carotid body a unique oxygen-sensing organ in comparison with other tissues [22].
Autonomic nerves play an important role in regulating the functions of the airways, including airway smooth muscle tone, mucus secretion, and blood flow. Afferent nerves in the airway are important with regard to airway defenses (cough), inducing reflex effects, and through the release of neuropeptides (neurogenic inflammation). Cholinergic nerves are the major bronchoconstrictor pathway through the activation of muscarinic receptors on airway smooth muscle. By contrast, adrenergic nerves have little direct control of airway smooth muscle, circulating E being more important in adrenergic regulation. A neural bronchodilator pathway is mediated by release of NO. Several neuropeptides are expressed in airway nerves and play a co-transmitter role in concert with the classical autonomic transmitters [23]. Autonomic nerve function is regulated primarily through reflexes initiated upon bronchopulmonary vagal afferent nerves [24].
During NREM sleep, there is a decreased chemosensitivity and apneas at the onset of the sleep phase [25]. During REM sleep, the decrease and variability of chemosensitivity, with a greater propensity to apneas and the decrease in the neural discharge to the respiratory muscles (except the diaphragm), are accentuated.
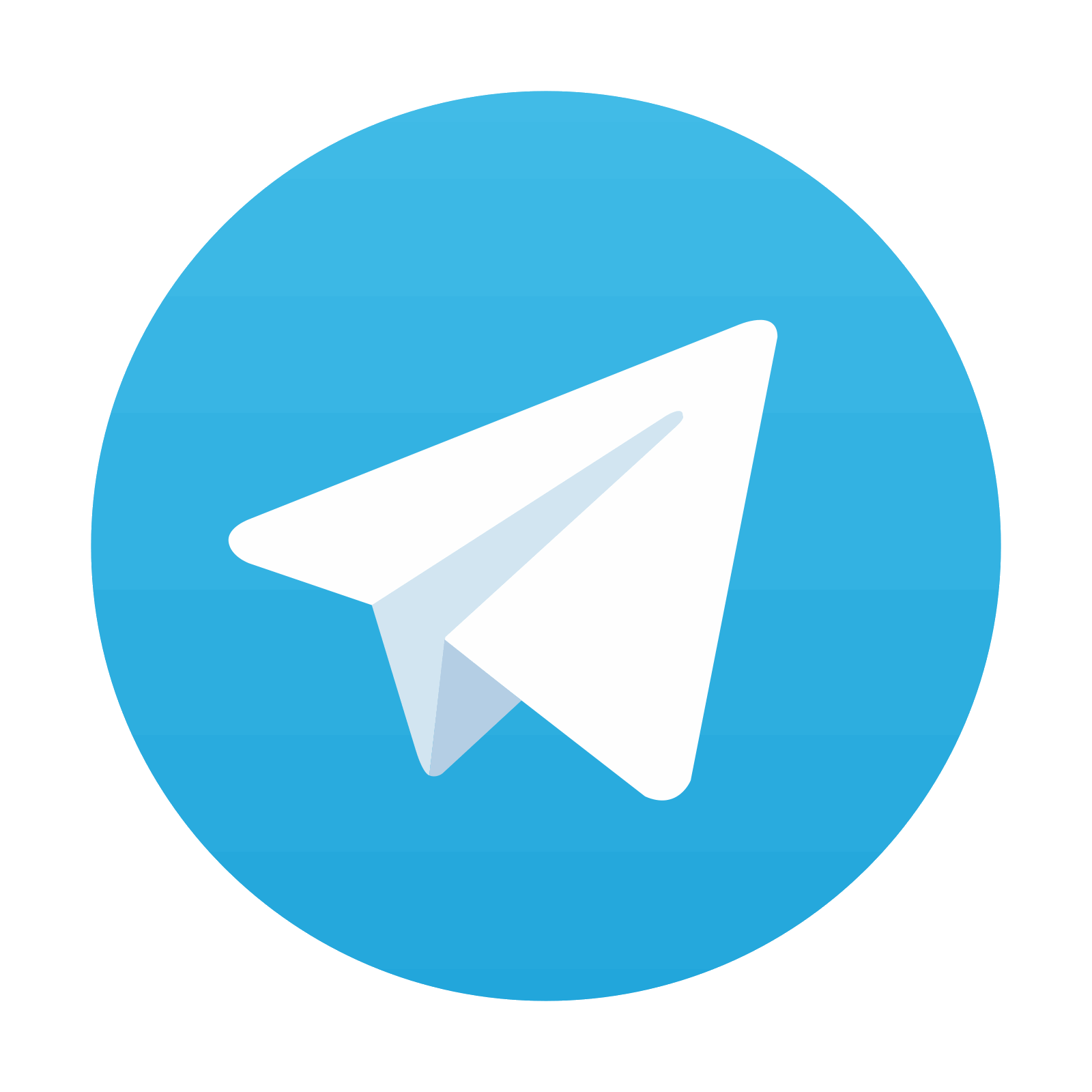
Stay updated, free articles. Join our Telegram channel
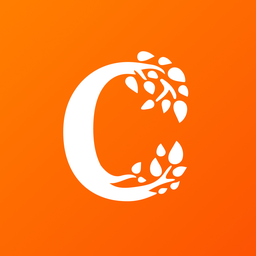
Full access? Get Clinical Tree
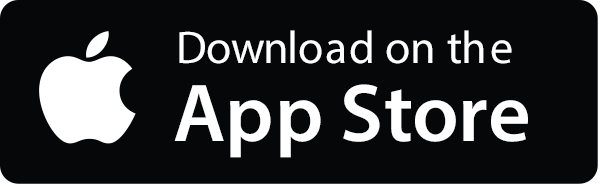
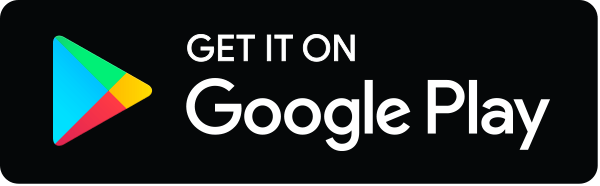