12 There have been four major advances in stereotactic surgery. The first occurred when the coordinate-based fixation systems used for standardized lesions in laboratory animals in the late 19th century were adapted for human clinical use. The second was the emergence of the arc-centered frame. The third was substitution of the stereotactic probe for the radiation beam. The fourth advance, still evolving, is incorporation of digital technologies into the stereotactic planning process. The interventions fueling this process were first computed tomography (CT) followed by magnetic resonance imaging (MRI) and the emergence of the modern personal computer. The computer changed the concept of stereotactic reference space. In traditional procedures, the working volume is defined by a fiducialized box. In contemporary neuronavigation, the walls of the box are reduced to a reference corner defined by the convergence of three perpendicular planes. Contemporary image fusion programs merge images from several modalities without requiring identical image planes. We commonly speak of frame-based and frameless stereotactic techniques. The distinction, however, is not completely clear. For the central nervous system (CNS), which is highly topographic and requires greater accuracy to target than a homogeneous organ such as the liver, invasive pinpoint fixation remains the gold standard. It can be argued that it makes little difference whether the patient is imaged in a frame or with surface fiducials: the target anatomy is still immobilized for treatment. The discomfort caused by a frame or a mask mold, however, is no trivial matter for patients. Because the head is more easily fitted in a frame than the trunk, traditional stereotaxis has mainly dealt with intracranial pathology. With the advent of superior imaging and computing capabilities and with a cultural preference for minimally invasive procedures, stereotaxis has broken out of the mold, literally and figuratively. Although the gamma knife unit (GKU) remains the gold standard for precision radiation treatment, it is a cranial setup based on the concept of the arc-centered frame. The linear accelerator (LINAC) has made it possible to adapt stereotactic radiation to the whole body. The newest technologies go beyond mere correlation of image, target, and treatment and incorporate reimaging capabilities activated by motion detectors to update the treatment plan in accordance with shifts in a patient’s position. In an ideal frameless stereotactic radiation system, a change in position is detected instantaneously and the target coordinates adjust accordingly. Immobilization of the patient is unnecessary. Unfortunately, the ideal system does not yet exist. The available systems that combine radiation and imaging capabilities are expensive and still measure delays in minutes. Some form of patient fixation or sedation is still required. For the foreseeable future these machines will not be cost competitive with fixed-frame techniques. With these limitations in mind, the first spinal frame for stereotactic radiosurgery was developed at the University of Arizona, closing the historical circle on frame-based stereotaxis. It is seldom remembered that stereotaxis has its roots in spinal applications. In the latter half of the 19th century, frames were designed for standardized lesions within the spinal cord of laboratory animals. As reported by Gabriel and Nashold,1 the first documented use of such a device was by Dittmar in Leipzig in 1873. Woroschiloff, a colleague of Dittmar, introduced a “myelotome” the next year to create reproducible lesions in the spinal cord of rabbits.1 In the strictest sense, these devices were not stereotactic devices because they were not based on a Cartesian three-dimensional coordinate system. The first truly stereotactic instrument for experimental use in the spine was introduced by Clarke in 1920.1 Like Woroschiloff’s device, Clarke’s instruments required clamps to be placed on the exposed lamina for skeletal fixation. The first clinical application of a cranial stereotactic frame was described by Spiegel and coworkers2 in 1947. The use of closed procedures on the spinal cord began in 1963 when percutaneous cordotomy was introduced by Mullan et al.3 The first time a stereotactic frame was used clinically for a spinal procedure was in 1965 when Rand (as reported by Gabriel and Nashold1) performed cryosurgery on the cervical spinal cord. This procedure and the subsequent work of Crue et al4 on trigeminal tractotomy in 1967 was achieved by caudal extensions to a head frame affixed to the skull. Likewise, Hitchcock5 in Edinburgh used a modified Leksell frame to perform a spinothalamic tractotomy. These head-frame extensions limited access to the juxtacranial cervicomedullary region. In 1972 Nadvornik (see Gabriel and Nashold1) revived and modified Woroschiloff’s technique with a device fixed to a lumbar vertebral arch through a laminectomy incision. In the early 1980s Cosman and coworkers6 tested a small stereotactic instrument that attached to the spinal lamina after a laminectomy and was used for myelotomy. In 1990 Heikkinen7 described a modified table-attached Laitinen head frame for spinal use. In 1993 Koutrouvelis and coworkers8 described a floor-mounted device called the PGK for CT-guided stereotactic discectomies. In the last few years, frameless stereotactic methods have been used for percutaneous pedicle screw fixation.9 In 1994 Lax et al10 described a body frame for fractionated radiotherapy. The next year Hamilton and colleagues11–13 presented a spinal stereotactic frame for LINAC-based single-fraction radiosurgery. The Cyberknife (Accuray Oncology, Sunnyvale, CA) was developed to achieve the dependability of the frame concept employing robotics and state-of-the-art computer image-guidance systems. In 1951 Leksell14 coined the term stereotactic radiosurgery. He proposed replacing the stereotactic probe in his own stereotactic system with a particle beam to cause an exact volume of tissue destruction without damaging the surrounding brain.15 This technique marked a significant departure from conventional radiotherapy where radiation is administered over a large field in a large, fractionated dose, depending on the differential radiosensitivity and repair capability of the neoplastic and normal tissues. Leksell wanted to deliver a large, single, precisely delivered dose that caused no adverse effects outside the target area. The elegance of the concept of radiosurgery is compelling. Based on the arc-center principle, several individually weak energy beams can be collimated to various widths and the volume of an effective dose shaped to spheres or near-spheres engulfing the target. In the simplest case, a spherical pathology is covered by an exact effective radiation shell as individual beams transect the target point at the center of the lesion. For a dumbbell-shaped tumor, two targets need to be determined. In theory, particle radiation is ideal for the purpose because the beams travel a finite distance, and the point of energy deposition, the so-called Bragg peak, is predictable and can be made to correspond to the depth of the lesion; however, particle accelerators and cyclotrons are large and expensive to install, and there are problems with covering irregular targets.16 Concise reviews of radiophysics are available elsewhere.16,17 Leksell abandoned the proton beam in favor of cobalt-derived electromagnetic radiation. He also abandoned the concept of a mobile beam source for a static solution where the patient is placed in the path of a predetermined beam cluster—the GKU, which was a direct adaption of the arc-center frame design. Computer-controlled precision robotics did not exist at the time, and the simultaneous delivery of all beams in an immobile device was simple and more reliable over time than mechanical orbiting devices. Today the GKU remains the gold standard for precision radiation delivery. Although the original concept was to use the GKU for functional neurosurgery, it was soon used to treat vascular malformations18 and tumors19 with considerable success. Stereotactic radiosurgery has now proven its usefulness in the treatment of functional disorders,20–22 tumors,23,24 metastases,25,26 and arteriovenous malformation.27–29 Because a GKU is expensive, heavy, and designed for cranial treatment alone, many centers choose to use a LINAC to achieve the same goals. The LINAC is a general-purpose radiation delivery machine that can be coupled with specific software and stereotactic hardware to deliver highly precise radiation. In a LINAC, the beam source, mounted on a gantry or on a robotic arm, orbits the patient. Compared with the GKU, the modern LINAC may be less accurate mechanically, but its overall treatment error approaches that of GKU.30 The LINAC, with its open constellation, has a significant advantage: it has opened the way for the extracranial application of stereotactic radiosurgery. In recent years, thanks to the relatively affordable and versatile LINAC, the spine has become accessible for radiosurgical treatment. In 1994 Lax and coworkers10 at the Karolinska Institute in Stockholm described a vacuum pad stabilizer for fractionated delivery of stereotactic radiation to extracranial targets. In 1995 Hamilton and colleagues11,13 at the University of Arizona described a skeletal fixation frame for spinal radiosurgery. The Cyberknife is a sophisticated frameless apparatus equally able to target the cranium or the spine because of its open configuration. Spinal tumors compose 15% of CNS neoplasms.31 Most of the tumors affecting the spine are metastases.32,33 In the spinal column, metastases greatly outnumber primary neoplasms. Typically, metastases to the spine are skeletal or epidural. Of the primary tumors, two thirds are extramedullary. In the United States, the annual incidence of spinal metastases is ~100,000. About 20,000 to 25,000 of these cancer victims will suffer epidural spinal cord compression as a manifestation of their metastatic disease. The rest will experience pain without a neurologic deficit. A minority benefits from traditional radiation, chemotherapy, or both. Often, vertebral metastases signal disseminated disease, and open surgery is no longer a feasible alternative. Following the complex form of the vertebrae, tumors that invade them also can assume complex shapes. This complexity complicates the dose-planning process.34 Undesirable radiation effects limit the volume and dose that can be administered to the CNS. The limits have been derived from animal studies and retrospective analyses of adverse clinical outcomes. Schultheiss et al35 reviewed radiation myelitis associated with various radiation regimens. Mathes and Alexander36 reviewed the basics of radiation injury. Although the position of white and gray matter is reversed in the spinal cord, the basic organization is the same for the brain and spinal cord. Consequently, the damage associated with radiation is quite similar. The overall cellular mechanism of radiation injury is not fully understood, although the common denominator is injury to deoxyribonucleic acid (DNA), either by direct disruption of bonds or by the formation of free radicals as the radiation interacts with water.36 In the spinal cord, demyelination and malacia are the dominant morphologic features. The blood supply to the spinal cord is provided by one anterior and two posterior spinal arteries, all relatively small. Vascular changes from hyaline degeneration and vasculitis to thrombosis and frank hemorrhage also occur. None of these changes alone is pathognomonic for radiation injury. The combination of vascular changes and demyelination, however, is characteristic of radiation myelitis. There are several theories of the pathogenesis of radiation myelitis.37 Some workers cite oligodendrocytic death or apparent injury to nutritive vessels.35 It can be assumed that radiation myelitis is a combination of primary radiation-induced oligodendrocytic death and secondary cell death caused by a disturbed blood supply. Astrocytic scarring from radiation myelitis and microglial phagocytosis also contribute to the cumulative damage. Radiation myelopathy with a latency of less than 6 months is rare in the spine. Spinal cord doses less than 50 Gy rarely cause radiation myelopathy. For a patient with malignant disease, the most common cause of myelopathy is progression of disease.35 The spinal cord is relatively more sensitive to large doses per fraction than other tissues.35 This discovery was empirical: Schultheiss et al35 compiled several cases of radiation myelitis after treating patients with doses of 40 Gy in fractions of 4 Gy. Commonly, a dose limit of 45 Gy in 22 to 25 fractions is recommended for the spinal cord. For myelopathy to occur at this treatment level, patients must harbor contributory disease with preexisting vascular compromise. Peters38 points out that the risk at this level is less than 1%, and just as one must guard against unnecessary overdosing, one also must not compromise tumor control from timidity or fear of litigation. Routine dosing below 45 Gy does not decrease the observed incidence of radiation myelitis because that dose lies on the flat portion of the dose-response curve.38 PRM5% is probably between 57 and 61 Gy, and PRM50% between 68 and 73 Gy. In each case, the risk must be weighed against the aim of treatment.36 An important issue in spinal stereotactic radiosurgery is the effect of radiation on bone. Most data stem from the treatment of pediatric tumors: It is known that radiation can cause hypoplasia of facial bones in children.39 Adult bone is more resistant, and necrosis is rare. The changes seen are vascular, reducing the bone’s ability to heal and to resist infection.36 Rat studies show a steep rise in spinal cord tolerance with dose fractionation. Tolerance also increases as the dose per fraction is reduced. Varying the dose rate and time interval over which a fractionated dose is delivered has the same effect as fractionation (Figs. 12-1 to 12-4). The tolerance of the spinal cord sets limits for hyperfractionation. If the interval is less than 6 hours,40 repair of radiation damages is incomplete and the risk of damage to normal tissue increases. Treatment time, the time over which the fractions are administered, influences tolerance in an intricate interplay with interfraction times. Increasing the interfraction time to longer than 24 hours does not increase tolerance, at least not if the overall treatment time is no longer than 6 to 8 weeks. Beyond this time, a second phase of late tissue recovery has been observed in animal models. This finding suggests that something could be gained by separating two fractions by perhaps months. Whether this mechanism exists in humans is unclear.40 As for treatment volume, the tolerance of the spinal cord decreases with increasing volume of exposure.37 For small volumes, animal data also suggest that the tolerance level of vascular structures is lower than that of white matter. FIGURE 12-1 Fractionation allows a greater total dose to be delivered without unwanted tissue damage. The tolerated dose is inversely related to the volume exposed. FIGURE 12-2 Altering fraction-dose and fraction-dose delivery time can increase the tolerable total dose beyond that available by fractionation itself. The interfraction time must be at least 6 hours; hyperfractionating at shorter intervals does not allow sufficient time for tissue repair. FIGURE 12-3 Fractionation allows an increase in the total dose that can be delivered without increasing the risk of unwanted tissue damage. FIGURE 12-4 Increasing the number of fractions and the interval between them increases the total dose that can be delivered without increasing the risk for tissue damage. Schultheiss and coworkers35 have used the SOMA (subjective, objective, management, analytical) scales to characterize the morbidity associated with radiation myelitis (Table 12-1). Myelography is either negative or shows slight swelling of the spinal cord. CT seldom provides useful information. MRI reveals edema of the spinal cord, which is hypointense on T1-weighted images and hyperintense on T2-weighted images. Cerebrospinal fluid (CSF) studies tend to be normal; sometimes the protein levels are elevated. Nerve conduction studies show reduced velocities concordant with myelin loss. There are three concepts of spinal stereotactic radiosurgery: bone screw fixation, contour mold fixation, and frameless stereotaxy. Bone screw fixation dates from 1874 to the apparatus of Woroschiloff designed for neurophysiologic animal research. The Hamilton-Lulu frame is based on traditional principles of rigid skeletal fixation. The frame is a rigid box, 200 × 50 cm long and 18 cm deep. The dimensions of the cradle are such that it fits in a standard scanner aperture of 56 cm. The x–y plane is determined by the CT scanner field guide light. The x–z plane is fixed by leveling the box with a high-precision machinist’s level. The two planes establish an orthogonal coordinate system. The origin of the coordinate system is the center of a calibration sphere placed as closely as possible to the intended treatment target. The patient is prone. The skeletal fixation is achieved by clamping the exposed spinous processes above and below the target area. Distally, the clamp can be replaced by bilateral pelvic fixation pins. For cervical and high thoracic targets, the proximal clamp can be replaced by a head ring. Once the coordinates for the target have been acquired from the CT images, the box is transferred to the LINAC treatment table and aligned using the LINAC guide lasers (Figs. 12-5 and 12-6).11–13,34 Based on a series of experiments using both phantom and ceramic targets embedded in animal cadavers, the measured stereotactic radiation treatment error with this technique is 2 mm. Phantom target experiments13 found a mean localization error of 1 ± 0.22 mm. The overall radiation treatment error was 1.4 ± 2 mm. Experiments with a porcine model preceding the human application41 verified the accuracy of the spinal frame concept and provided data for the optimal placement of clamps to eliminate respiratory motion.
Spinal Stereotactic Radiosurgery
History of Spinal Stereotaxis
History of Radiosurgery
Neoplastic Disease in the Spine
Radiosensitivity of the Spinal Cord
Fractionation
Diagnostic Evaluation for Radiation Myelitis
Techniques of Stereotactic Radiosurgery
Bone Screw Fixation
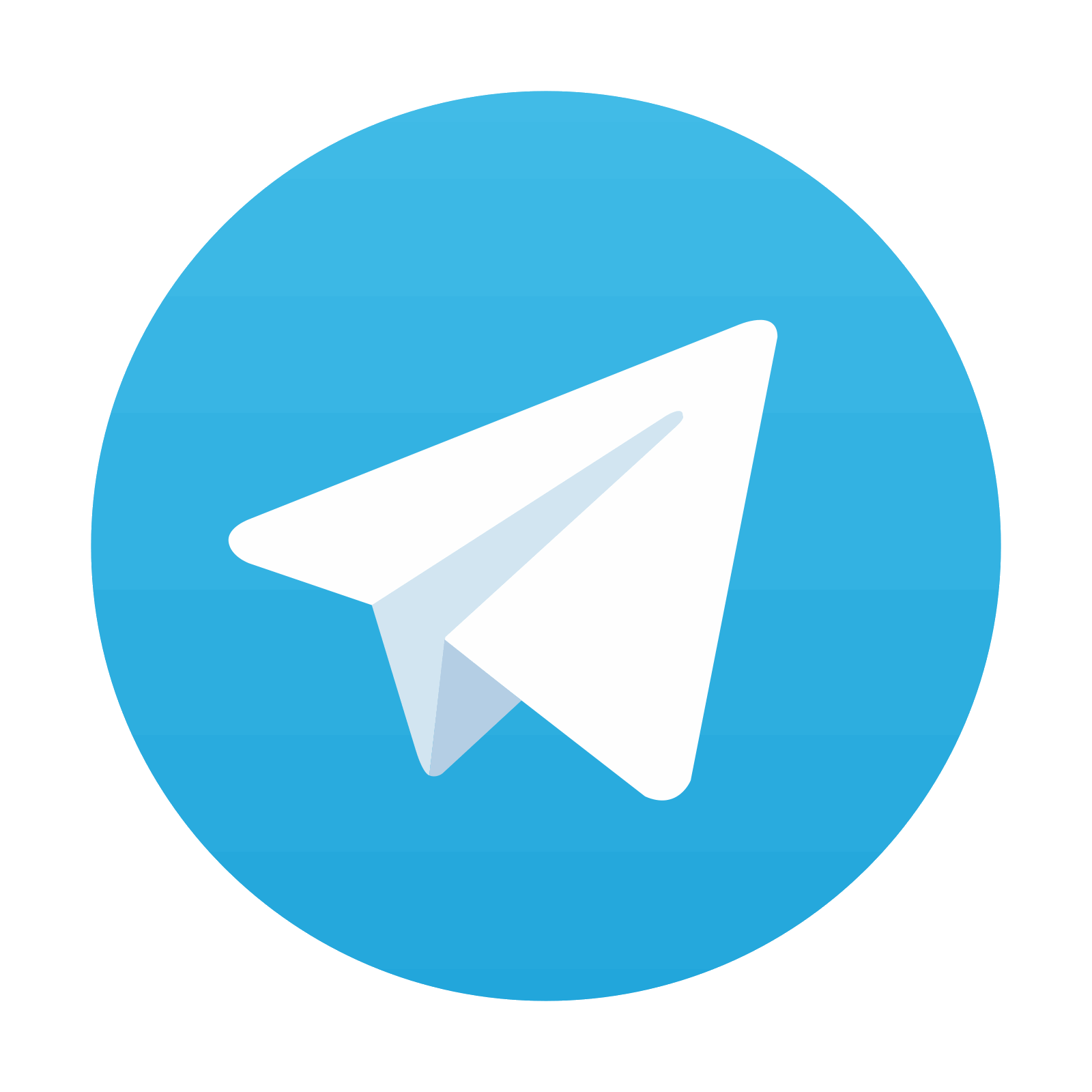
Stay updated, free articles. Join our Telegram channel
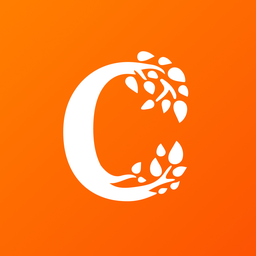
Full access? Get Clinical Tree
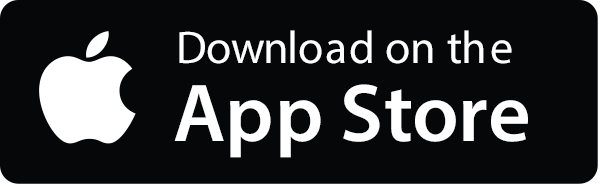
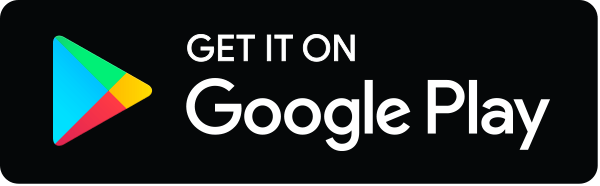