Figure 57.1. Coronal view illustrating meningeal layers and common sites of central nervous system infections [3].
57.3 Pathogenesis of Bacterial CNS Infections
Clinical complications of bacterial CNS infections are largely mediated by host immune responses and leukocytes. An estimated 50% of neuronal cell loss occurring in the context of Streptococcus pneumoniae meningitis is the result of bacterial toxins (pore-forming pneumolysin, hydrogen peroxide); the other half is mediated by the inflammatory process [9-11]. Endogenous inflammatory mediators, such as interleukin IL-1, IL-6, and tumor necrosis factor TNF-α, which are part of the host immune response, contribute to neurologic injury by stimulating migration of neutrophils into the CSF. Proinflammatory cytokines (IL-1a, IL-1b, TNF-α, IL-6, granulocyte-macrophage colony-stimulating factor), produced by these invading leukocytes, activated endothelial cells, astrocytes, and perivascular macrophages, regulate brain inflammation by altering brain endothelial cell junction complexes, which disrupts the BBB and results in the development of vasogenic brain edema, increased intracranial pressure, and disruptions in cerebral blood flow [12-15]. Intercellular adhesion molecules, vascular cellular adhesion molecules, and selectins alter tight junction complexes by regulating leukocyte and endothelial cell interactions, and leukocytes themselves increase vascular permeability through interactions with endothelial cells and release of proinflammatory mediators [15-17]. Finally, chemokines and chemoattractant cytokines such as IL-8, monocyte chemoattractic protein-1, and chemokine ligand (CCL2) are proinflammatory mediators that selectively drive leukocytes into brain parenchyma and can regulate BBB permeability by expressing specific receptors on brain endothelial cells and by producing other proinflammatory agents [18].
Stimulation of the host immune response depends on components of the bacterial cell wall and lipopolysaccharide surface proteins [19]. Because levels of complements, immunoglobulins, and polymorphonuclear leukocytes are lower in the CSF than the serum, opsonic activity is inferior, and therefore bactericidal as opposed to bacteriostatic agents must be used to control infection [20,21].
There are also factors limiting excessive host immune responses. The TNF-related apoptosis-inducing ligand (TRAIL) appears to modify the inflammatory response and reduce neuronal cell death. Soluble TRAIL levels are elevated in the CSF of patients with bacterial meningitis [22]. In experimental meningitis, using intrathecal administration of pneumococcal cell wall (a TRAIL receptor ligand), TRAIL deficient mice had protracted inflammation, clinical impairment and increased apoptosis in the hippocampus. These changes were reversed by administration of recombinant TRAIL (rTRAIL) into the subarachnoid space of TRAIL-/- mice or by transplanting TRAIL-expressing bone-marrow cells into a chimeric mouse model. Therefore, TRAIL is an anti-inflammatory cytokine that limits the lifespan of activated leukocytes, mediates neuronal injury in meningitis, and may be useful as a therapeutic agent to reduce the acute inflammatory response and improve outcomes in invasive CNS infections [23].
Removing cytokine and chemokine activity with neutralizing antibodies to cytokines and chemokine receptor antagonists, respectively, has been shown experimentally to reduce brain edema formation. This may represent a new strategy for treating vasogenic brain edema, which causes significant morbidity in CNS infections [18,23,24]. Blockade of TNF-α, which contributes to cochlear injury, reduced postmeningitic hearing loss, and cochlear injury after Streptococcus pneumoniae meningitis [25]. Brain-derived neurotrophic factor, which has marked antiapoptotic effects in hypoxic ischemic injury, significantly reduced the extent of brain cell injury in experimental meningitis [26]. These therapies may become useful as adjunctive treatments for bacterial meningitis.
Also of importance is the blood-CSF barrier. The choroid plexi in the ventricles are perfused by unique fenestrated capillaries. The barrier function is produced by tight junctions between ependymal cells separating these capillaries from the ventricular cavity. The BBB and blood-CSF barrier have different physiology, but they both restrain CNS drug distribution [27-29]. Altered permeability of these barriers during meningeal inflammation can significantly increase drug penetration into the brain parenchyma and CSF, especially those that are poorly lipid-soluble [30].
57.4 Pathogenesis of Encephalitis
Encephalitis is an acute inflammation of the brain parenchyma. The most common causative agents are viruses including mumps, herpes simplex virus (HSV), cytomegalovirus (CMV), varicella zoster virus (VZV), enteroviruses, togaviruses (eastern and western equine viruses), and lymphocytic choriomeningitis virus. Recently West Nile virus (WNV) and monkeypox in North America, and Chandipura viruses in the developed world have emerged as important causes of viral encephalitis [31]. Also, encephalitis caused by CMV, Epstein-Barr virus (EBV) and human herpes virus (HHV)-6 has increased with increasing populations with immunocompromised states, including HIV, organ transplantation, and cancer chemotherapy [32]. Immune-mediated conditions associated with encephalitis include acute disseminated encephalomyelitis, paraneoplastic limbic encephalitis, and voltage gated potassium channel limbic encephalitis [32]. Other microorganisms that can cause encephalitis include protozoa, such as Toxoplasma gondii, and bacteria, such as Listeria monocytogenes and Mycobacterium tuberculosis.
Viruses enter the CNS via reactivation of latent virus within the CNS or by viremia, followed by migration across the blood-brain barrier. Blood transfusions and donated organs are also increasingly recognized as sources of transmission [31]. Virus enters brain cells by adhesion to specific cell surface receptors on glia and neurons. Once inside the cell, the virus expresses proteins which inhibit apoptosis and complement opsonization which allows the virus to prevent detection by glia. Pathology occurs due to viral destruction of cells, para- or post-infectious inflammation, and by immune mediated responses [32]. Although neurons are primarily affected, blood vessels may be involved, causing vasculitis. Other associated features include meningitis, myelitis, radiculitis, and demyelination. Cerebral edema and raised intracranial pressure can result due to induction of perivascular lymphocytic inflammation, primarily in the gray matter and at the gray-white matter junction [33].
57.5 Clinical Evaluation of Patients With Suspected Central Nervous System Infection
Critical information leading to etiologic diagnosis can be obtained from a thorough examination of clinical features [34]. Identification of infectious agents in CNS is highly dependent on the CSF analysis, and occasionally serum or biopsy data. Neuroimaging also plays an important role in diagnosis and therapeutic decision-making.
57.5.1 Clinical Presentation
Acute Septic Meningitis
Acute bacterial meningitis is a neurologic emergency, with mortality and morbidity rates as high as 25% and 60% respectively, despite improvements in critical care [35,36]. In adults, the classic triad of fever, neck stiffness, and altered mental status has a low sensitivity for diagnosis of bacterial meningitis. A Dutch study of 696 episodes of adult community-acquired acute bacterial meningitis confirmed by CSF cultures reported the full triad in only 44% [37]. More sensitive was the finding of at least two of the four symptoms of headache, fever, neck stiffness, and altered mental status which occurred in 95% of episodes. On admission, 14% of patients were comatose, and 33% had focal neurologic deficits. A retrospective French study investigated factors distinguishing between bacterial and viral meningitis in 90 patients with confirmed bacterial meningitis and 54 patients with untreated viral meningitis [34]. The presence of one of four findings at admission, among altered consciousness, seizures, focal neurologic findings or shock, and CSF absolute neutrophil count above 1,000/mm3 was predictive of bacterial meningitis. CSF glucose less than 2 mmol/l and CSF protein above 2 g/l did not distinguish between bacterial and viral meningitis.
It is important to remember that patients who are elderly, immunocompromised, or previously treated with antibiotics may not manifest a febrile response. The combination of altered mentation and fever should alert the clinician to the possibility of meningitis in any elderly patient [38]. Other common findings are nuchal rigidity (30%) and focal cranial nerve palsies, including gaze paresis caused by hydrocephalus [39]. Seizures are reported to occur in 5% to 28% of meningitis cases and are a sign of cortical irritation, possibly due to a cortically based complication such as empyema, stroke, or venous thrombosis [40-42]. Seizure activity is an independent predictor of mortality [42]. In a review of 103 episodes of acute bacterial meningitis in adults, 34% of patients who had seizures died compared with 7% without seizures. Decreased level of consciousness on presentation is also predictive of mortality (26% versus 2%). Altered mental status is common in bacterial meningitis. The average Glasgow Coma Scale (GCS) score on admission is 10-11, of which 10-20% of patients are comatose [37]. It should be remembered that recovery is possible after prolonged deep coma, depending on the degree of structural brain pathology. Coma caused by diffuse cerebral edema can lead to cerebral herniation. Coma may also be caused by hydrocephalus and by multiple cerebral infarcts secondary to vasculitis [43].
Raised intracranial pressure is likely common in bacterial meningitis, but not frequently monitored. Intracranial hypertension in meningitis can occur due to cytotoxic edema, vasogenic edema and communicating hydrocephalus [44]. From van de Beek et al., mean opening pressure at time of diagnostic lumbar puncture was significantly elevated at 37 mmHg and was greater than 40 mmHg in 39% of patients, many of whom were comatose [37]. From a small Swedish study of patients with bacterial meningitis and GCS <8, 60% had raised intracranial pressure (ICP) on admission and 14/15 developed intracranial hypertension [45]. The mean initial ICP was higher in non-survivors (46 mmHg) compared to survivors (20 mmHg). No randomized controlled trials have investigated the potential benefit of ICP monitoring in comatose patients with CNS infeciton. Elevated ICP induces secondary brain injury by reducing cerebral perfusion pressure (CPP) leading to ischemia and by causing brain shifts leading to herniation syndromes.
Abnormal cerebral blood flow (CBF) autoregulation is common in patients with bacterial meningitis [46]. This implies that the cerebral circulation is directly dependent on blood pressure and therefore vulnerable to ischemia under conditions of low systemic blood pressure. Cerebral vasculopathy has also been documented in patients with bacterial meningitis which increases risk of ischemic stroke [47]. Other vascular complications of meningitis are cerebral venous thrombosis, venous infarction and intracerebral hemorrhage. Arterial or venous vascular complications are reported in up to 20-30% of patients [48].
A few rare manifestations of meningitis may provide clues to the causative organism: petechial or purpural rash in meningococcal meningitis, ataxia and labyrinthitis in Haemophilus influenzae meningitis, and cough, weight loss, night sweats, and cranial nerve deficits in tuberculosis meningitis (TBM). Systemic complications in bacterial meningitis include septic shock in 12%, pneumonia in 8%, and disseminated intravascular coagulation in 8% of cases [41]. Mortality is often related to systemic complications rather than neurologic causes, especially in the elderly, emphasizing the importance of critical care management of these patients. Risk factors for unfavorable outcome from 696 episodes of adult bacterial meningitis in the Netherlands treated between 1998 and 2002 included the following [37]:
- Advanced age.
- Presence of osteitis or sinusitis.
- Absence of rash.
- Low admission Glasgow Coma Score.
- Tachycardia.
- Positive blood culture.
- Elevated erythrocyte sedimentation rate.
- Thrombocytopenia.
- Low CSF white cell count.
The major risk factors for mortality are: seizure activity, decreased level of consciousness (LOC), Inadequate antibiotic therapy and gram-negative meningitis.
Encephalitis
The clinical scenario of encephalitis is a febrile patient who presents with altered mental status or other signs of diffuse cerebral dysfunction. A prodrome with fever, headache, myalgia, and mild respiratory infection is often present. Changes in level of consciousness with focal neurologic deficits may follow. Seizures are common, both focal and generalized; occurrence depends on the etiological agent [49,50]. Some characteristic presentations are parotitis associated with mumps and herpetic rash with Herpes simplex encephalitis (HSE) although the latter is rare. Immunocompromised patients with brainstem HSE may present with cranial nerve palsies such as diplopia, dysarthria, and ataxia [51,52].
HSE is the most important form of treatable encephalitis. The clinical picture is altered consciousness, memory loss, personality change, and confusion or olfactory hallucinations, following a prodrome of headache and fever [53]. About two thirds of patients have seizures, often focal motor seizures affecting the face and upper extremities due to frontal and temporal lobe involvement. Focal neurologic signs like hemiparesis and aphasia are more common with HSE than other encephalitides. HSE is a medical emergency, and it is associated with a high mortality rate and numerous sequelae. Over 20% of survivors have severe disability which includes cognitive and behavioral disorders, seizures, and postinfectious encephalomyelitis [54,55].
Most cases of adult HSE appear to be caused by reactivation of HSV-1, either in the trigeminal ganglion or latent virus in the brain itself [56] although more recently it is unclear if HSE represents primary or recurrent infection and strains of HSE and cutaneous herpes simplex may be different. HSE has no identified triggering factors and although immunosuppression is associated with more aggressive disease and worse outcome, it does not predispose patients to the disease [57]. HSV-2, which causes genital herpes in adults, accounts for approximately 10% of HSE, mostly in neonates and the immunocompromised. Most cases of recurrent meningitis (previously called Mollaret’s meningitis) are thought to be caused by HSV-2 [50].
West Nile virus encephalitis (WNVE), caused by a single-stranded RNA virus of the Flavivirus family appears to only cause severe human disease in the United States and Israel [58]. The disease usually affects persons over age 55, and has a 15% mortality rate [59,60]. Neuroinvasive WNV only occurs in an estimated 1% to 2% of infections, but the risk of encephalitis increases with age and in immunocompromised states [49]. Without encephalitis, WNV represents a flu-like illness. Signs of encephalitis are typical: fever, fatigue, nausea/vomiting, headache and altered mental status. WNVE presents with delirium in 38%, stupor in 27% and coma in 22% [61]. Other neurologic features include movement disorders, sometimes with Parkinsonian features, postural tremor, and myoclonus and cerebellar signs [61-63]. Seizures are infrequent. WNV can cause an acute flaccid limb paralysis in 10% of neuroinvasive cases, caused by infection of the anterior horn cells of the spinal cord, causing profound weakness and respiratory failure [64]. This poliomyelitis-like illness also occurs in other flavivirus encephalitides (Japanese encephalitis virus and St. Louis encephalitis virus) and enteroviruses [61-65]. The mortality in WNV is caused by respiratory failure in half of such cases. Morbidity is high, due to long-term limb weakness and functional disability [64]. Ophthalmologic disease, such as chorioretinitis and vitritis, and rhabdomyolysis are associated findings [66,67]. Pediatric WNV has similar manifestations and infection rates to adults and accounted for 12% of all neuroinvasive cases in the United States in 2004 [68,69].
Brain Abscess
Brain abscesses are focal, purulent infections of brain parenchyma. Site-specific focal neurologic deficits, such as aphasia and weakness are common although signs of increased intracranial pressure may be the only presenting feature [70]. Fever is less common than in meningitis and when neck rigidity is present (25% of cases) it may indicate associated meningitis [38]. Seizures occur in up to 40% of cases and are often generalized tonic-clonic [71]. The route of transmission is usually contiguous spread from a local primary focus (paranasal sinusitis, otitis media, mastoiditis, penetrating head trauma (10%)). Twenty percent of cases spread hematogenously from either a pulmonary source (bronchiectasis, lung abscess), cardiac source (heart valve – infective endocarditis), or conditions with right to left shunt. The mouth, skin, and gastrointestinal tract may also be sources. In cases of hematogenous seeding, multiple abscesses are seen in 20%. Polymicrobial infections are not uncommon. Staphylococcus pyogenes is common in penetrating skull injuries. Organisms to consider in immunocompromised patients are Listeria, mycobacteria, fungi, and parasites. The important complications from abscess to watch for include strokes caused by cortical thrombophlebitis, rupture of abscess into ventricle or subarachnoid space causing meningitis or ventriculitis and increased intracranial pressure. The mortality from brain abscess is 10% overall.
Cranial Epidural Abscess
Cranial epidural abscess is an extra-axial infection occurring in the virtual space between the dura mater and the skull. It is most often located in the frontal region, and presents with headache, fever, and nausea, but rarely focal neurologic signs. Spread of infection is limited by tight adherence of dura to the overlying skull. These infections are usually related to trauma and neurosurgery, but can occur secondary to meningitis, sinusitis, and other extra-cranial sources. The most common infectious etiologies are streptococci, staphylococci, and anaerobes, with many infections being polymicrobial [72,73].
Subdural Empyema
Subdural empyema occurs in the potential space between the dura mater and the arachnoid, most commonly over the cerebral convexity. Unlike epidural infections, subdural empyema can spread more diffusely and cause inflammation of the brain parenchyma, edema, mass effect, elevated intracranial pressure, septic thrombophlebitis, and venous infarction [72,74]. Findings are therefore more severe including altered level of consciousness, focal neurologic deficits, and seizures. The sources of infection are the para-nasal sinuses, hematogenous spread via emissary veins in the subdural space, and extension of an epidural abscess into the subdural space [72,75].
Infratentorial empyema is a rare complication of bacterial meningitis. It presents as subacute meningitis with neck stiffness and decreased consciousness and should be suspected in a patient with recent history of otitis, sinusitis, or mastoiditis (especially after surgery) and findings suggesting meningitis and a posterior fossa lesion [76]. The preferred method of diagnosis is MRI with diffusion-weighted imaging (DWI) based on several reported cases of delayed diagnosis with CT which failed to visualize the lesions. DWI can distinguish subdural empyema from reactive subdural effusion [77]. The high mortality rate of this condition (34%) is due to its subdural location, hydrocephalus, and delayed detection. The management is antimicrobial therapy and a low threshold for neurosurgical exploration.
Ventriculitis
Ventriculitis is an infection of the ventricular system of the brain. The ventricles act as a reservoir of infection and inflammation, which causes blockage of CSF outflow tracts and hydrocephalus [78]. Ventriculitis complicates meningitis in 30% of adult cases, and up to 90% of neonatal cases [74]. It is clinically important when inflammation and bacterial load in the ventricles exceed those in the paraspinal space [78]. It is often a late complication of meningitis, when patients fail to improve with conventional therapy, but it can occur as a primary process [79,80]. Ventriculitis is frequently associated with intracranial devices, namely a CSF shunt or external ventricular drain (EVD). The risk of ventriculitis or meningitis with an EVD in place is most commonly reported in the 10% to 15% range, and depends on the type of EVD, insertion technique, management, and duration [81-84]. Blood in the CSF is a significant risk factor for ventriculostomy-related infections, and contributes to the high incidence of these infections in aneurysmal subarachnoid hemorrhage patients (10%) and patients with intraventricular hemorrhage (IVH) (13.7%) who have EVDs [83-85]. Other significant risk factors for infection are protocol violations in EVD care and CSF leaks [86]. Infection is reported to occur in 8% to 40% of patients receiving a CSF shunt, most within the first month after implantation [72]. Common pathogens causing EVD and CSF shunt infections are gram-positive organisms, such as Staphylococcus epidermidis and Staphylococcus aureus. Up to 25% of infections, however, are caused by gram-negative organisms, such as Escherichia coli, Klebsiella species, Acinetobacter, and Pseudomonas species [72].
57.5.2 Cerebrospinal Fluid Analysis
Diagnosis and management of CNS infection largely relies on CSF analysis. It is important to obtain CSF access as soon as it is safe. Concerns about uncal or cerebellar tonsillar herniation and the need to initiate empiric antibiotics emergently are often competing demands. The literature supporting necessity of doing a head CT scan prior to lumbar puncture (LP) is minimal while the evidence in favor of not delaying antibiotics in suspected meningitis is significant. Unfortunately the availability of CT frequently delays starting antimicrobial therapy [87]. In van de Beek’s study of 696 episodes of community-acquired acute bacterial meningitis [37], CT was performed before LP in 48%, and in 63% of subjects presenting with impaired consciousness or neurologic deficits. In two thirds of subjects who had a CT scan, antimicrobial therapy was not started until after CT results were obtained. In many countries it is common practice to perform a head CT before performing LP in patients with suspected meningitis, due to a perception that this is a standard of care or because of fear of litigation [88]. In fact, only 1-2% of patients with meningitis herniated, and a much smaller percentage do so within hours of LP [44]. A normal CT does not eliminate risk for herniation. Criteria for performing head CT to assess risk for cerebral herniation prior to CSF access are the following [89,90]:
- Immunosuppression (to rule out Toxoplasma encephalitis or lymphoma).
- Significant alteration in mentation or comatose patient.
- Focal neurologic deficits.
- Seizures.
- Papilledema, poorly reactive pupils, or ocular palsies.
- Bradycardia or irregular respirations.
- Sedation or muscle paralysis.
In a prospective study of 301 adults with clinically suspected meningitis, 78% of subjects underwent CT before LP [88]. Of these, 24% had an abnormal finding and 5% had mass effect. Abnormal findings on CT were associated with the following clinical features at baseline:
- Age greater than 60 years.
- Immunocompromised state.
- History of a CNS lesion.
- History of seizures within 1 week before presentation.
- Abnormal level of consciousness.
- Inability to answer two consecutive questions correctly or follow two consecutive commands.
- Focal neurologic deficits including gaze palsy, abnormal visual fields, facial palsy, arm drift, leg drift, and abnormal language.
Of the 96 subjects who had none of these findings, only three had abnormal findings on CT and underwent LP without subsequent herniation (negative predictive value of 97%). The delay from admission to time of lumbar puncture in patients who first underwent CT resulted in a greater delay in administering empiric antibiotic therapy in this group [88]. It is well-described that the diagnostic sensitivity of CSF is not decreased by delaying LP by 1 or 2 hours after starting antibiotics [91]. If available, CSF counterimmunoelectrophoresis, CSF latex agglutination and coagulation tests detect common bacterial antigens in 70-100% of cases [92].
It is recommended that in all patients with clinical signs suggestive of increased ICP, even without abnormalities on head CT, one must exercise caution in performing an LP, or use a small 22- or 25-gauge needle to remove the smallest amount of CSF possible and minimize CSF leakage [89]. In a review of lumbar puncture associated with brain herniation in acute bacterial meningitis, the incidence of cerebral edema with brain herniation was 5%, accounting for approximatetly 30% of the mortality [93]. Many such reports describe a temporal association between the LP and the herniation event although herniation may also occur gradually due to ongoing CSF leakage from the LP site or due to cerebral vascular engorgement. The best clinical indication to delay the LP are clinical signs of ‘‘impending’’ herniation rather than CT findings. Aggressive treatment of herniation has been reported to produce good outcomes. Therefore, a high-risk patient should have blood drawn for culture and empiric antibiotic therapy initiated prior to CT.
Typical CSF findings in various CNS infections are presented in Table 57.1. Gram’s stain of CSF will show organisms in 60% to 90% of bacterial meningitis cases and cultures are positive in 80% [38]. Most patients will have a CSF white blood cell count greater than 100 WBC/mm3 in 90% of cases and greater than 1,000 WBC/mm3 in 60%.
Infection | Cell count (cells/mm3) | Glucose | Protein |
Bacterial meningitis | Elevated (100-5000) PMNs predominate | Decreased | Elevated |
Viral meningitis | Elevated (10-500) Lymphocytes predominate | Normal | Elevated |
Fungal meningitis | Normal to elevated (0-500) Lymphocytes predominate | Normal to decreased | Elevated |
Tuberculous meningitis | Normal to elevated (0-1000) Lymphocytes predominate | Decreased | Elevated |
Brain abscess | Normal to elevated (0-500) Mixed differential | Normal | Elevated |
Ventriculitis | Elevated (100-5000)* PMNs predominate | Decreased | Elevated |
Table 57.1. Cerebrospinal fluid findings in CNS infections.
* Note: in post-neurosurgical patients, or patients with a ventriculostomy, the CSF cell count may be elevated as a result of surgical manipulation and inflammation. Reduction in CSF glucose may be a more sensitive indicator of infection;
PMNs = Polymorphonuclear leukocytes.
CSF glucose levels are less than 40 mg/dl in 50% to 60% of patients. The CSF to serum glucose ratio of less than 0.4 is 80% sensitive and 98% specific for bacterial meningitis in children over 2 months of age [92]. Decreased CSF glucose is also seen in fungal, tuberculous, or carcinomatous meningitis. The CSF protein level is the most resistant to rapid change after starting treatment; levels remain elevated for 10 days or longer [94]. The CSF findings in subdural empyema and epidural abscess typically show a polymorphonuclear pleocytosis (10-500 WBC/mm3), normal glucose, increased protein, and often negative Gram’s stain and culture [71]. Blood cultures are positive in up to 50% of cases of meningitis [72]. Hyponatremia is common in both meningitis and encephalitis and is caused by the syndrome of inappropriate antidiuretic hormone (SIADH). CSF lactate concentrations are non-specific for suspected community-acquired bacterial meningitis, but may be useful for diagnosis of post-neurosurgical meningitis where a CSF lactate concentration cut-off of 4 mmol/l was superior to the CSF:serum glucose ratio [92,95]. This cut-off had a sensitivity of 88%, specificity 98%, positive predictive value 96% and negative predictive value 94%. Routine use of latex agglutination tests for bacterial antigens is not in common use because it does not appear to modify the decision to administer antimicrobials. Additionally, false-positive results have been reported [92]. They may be useful for patients pretreated with antibiotics with negative Gram’s stain and CSF cultures. Bacterial DNA detection by polymerase chain reaction (PCR) for common meningeal pathogens has relatively limited availability, but should increase with further refinements which improve sensitivity and specificity [92]. Broad-range real-time PCR and
DNA sequencing for bacterial meningitis detects virtually all pathogenic bacteria and has a sensitivity between 86% to 100% and specificity of 98% when compared with culture [96,97].
Laboratory diagnosis of tuberculous meningitis (TBM) remains challenging. The typical CSF profile is a lymphocytic predominant pleocytosis with elevated protein and low glucose, but has many exceptions, especially in HIV-positive patients [98-100]. The acid-fast stain for tuberculosis (TB) has sensitivity as low as 30%, which can be improved to about 80% with repeat CSF sampling and meticulous examination [72]. Culture, the gold standard, is only positive in 40% to 70% of cases and, even with fully automated liquid culture systems, takes from 1 to 3 weeks to a positive result. Several mycobacterial antigen and antibody kits have been designed but lack adequate sensitivity and specificity to gain wide acceptance [98,101,102]. CSF adenosine deaminase activity (ADA), a bio-marker, is elevated in TBM and in developing countries the CSF ADA activity at a cut-off value of 6.97 IU/l has a reported sensitivity and specificity of 85% and 88%, respectively, for distinguishing TBM from non-TBM or nonmeningitis [103]. CSF ADA levels are also elevated in conditions such as lymphoma and sarcoidosis. Molecular diagnosis with commercial nucleic acid amplification tests for diagnosis of TBM have a sensitivity of 60% to 83% and specificity of 98% to 100% [102,104]. Real-time PCR is fast and has greater sensitivity than conventional PCR, but still cannot be used reliably to rule out TBM [105]. Nucleic acid amplification tests, if available, should be the first-line test to rule in TBM due to their high specificity [105]. Radioimmunoassay detection of interferon-gamma release measures a major mediator of the immune response in TB, affecting macrophage stimulation and lymphocyte Th1 transformation [106]. Interferon-γ assays in CSF were compared with PCR for diagnosis of TBM and had a higher sensitivity (70% versus 65%) with a specificity of 94% [107]. Combination of PCR and interferon-γ improved sensitivity to 80% and the incremental value of test combinations such as these shows promise, although further prospective studies should be performed. Currently, careful bacteriology is as good as or better than molecular methods, although the latter are sensitive for longer when anti-TB drugs have been started [103,108].
Acute-phase reactants have been examined for their usefulness in diagnosing acute bacterial meningitis, but appear to have limited usefulness in the intensive care unit. Elevated procalcitonin (PCT) concentrations can differentiate between bacterial and viral meningitis in children (cut-off 0.5 mg/l; sensitivity 94%; specificity 100%) and adults (cut-off 0.2 ng/ml; sensitivity and specificity up to 100%) [109-111]. Of 48 adult patients with acute bacterial meningitis and a serum PCT level on admission above 0.5 ng/ml, serum PCT levels decreased rapidly (from 4.5 to 2 mg/ml) (within 24 hours) with appropriate antibiotic treatment, suggesting this test may replace repeat lumbar puncture at 48 to 72 hours to assess antibiotic therapy [112]. Alternatively, in patients with ventriculitis and a ventricular catheter, PCT levels usually remain within the normal range, even with positive bacterial cultures [113,114].
Nosocomial EVD-related infections are difficult to diagnose because of lower diagnostic value of CSF values, less specific clinical signs of infection, and slow-growing and fastidious organisms [115]. Broad-range real-time polymerase chain reaction (RT-PCR) may have a role in producing faster diagnosis (about 4 hours) with probable increased sensitivity over cultures after antibiotic treatment and ability to detect bacteria, especially gram-negatives that are difficult to isolate [96]. Disadvantages of PCR include inability to identify antibiotic sensitivities, false negative results, and contamination issues. The ratio of leukocytes:erythrocytes in CSF divided by leukocytes: erythrocytes in peripheral blood (called the cell index) has been used to detect EVD-related infection in patients with IVH [116]. At IVH onset, the CSF relationship of leukocytes to erythrocytes should be equal to that in peripheral blood; the cell index should be 1. In 7/13 patients with definitive ventriculitis, the cell index showed a significant rise 3 days before the conventional diagnosis of catheter-related ventriculitis. Antimicrobial therapy led to a rapid decrease of both the cell index and usual infection parameters [116].
Viral CNS infection is associated with normal-to-mild ICP elevation, CSF lymphocytosis (neutrophils if tested early), WBC counts of 50/mm3 to 2000/mm3, normal glucose, and elevated protein [71]. HSV-1 encephalitis is typically associated with hemorrhagic CSF and very high CSF red cell count. CSF culture and serologic testing of acute and convalescent serum may be beneficial. For HSE, the sensitivity and specificity of PCR in CSF are 98% and 94%, respectively. One third of cases remains PCR-positive long after starting acyclovir treatment [117]. It is recommended to repeat LP at 24-48 hours and continue treatment for 10 days, due to the possibility of false negative PCR for HSV with early testing if features are consistent with HSE [118,119]. PCR has good specificity for CMV (95%) and Toxoplasma (100%), but lower sensitivity (79% and 42%, respectively) [71]. In patients with suspected enteroviral meningitis, enteroviral RT-PCR is more sensitive than viral culture for detecting enteroviruses (sensitivity 86% to 100%; specificity 92% to 100%) [120]. The diagnosis of most important viruses, as well as Mycoplasma pneumoniae can be determined by detection of IgM (and IgG) antibodies in CSF (and relative to serum) by an enzyme-linked immunosorbent assay. Oligoclonal bands may distinguish inflammatory from noninflammatory causes of encephalopathy. Anti-HSV-1 antibody in CSF can be detected at 10 days, with 50% sensitivity [32]. Immunocompetent patients should be initially tested with PCR for HSV, VZV (both potentially treatable with antiviral therapy), and enterovirus [32]. For immunocompromised patients, EBV and CMV PCR should be added and HHV-6 and HHV-7 considered. Other PCRs to send depend on clinical indication. Quantitative PCR (comparing virus in CSF with that in blood) may be required to determine the significance of a positive PCR assay, especially in HIV patients who may carry infected lymphocytes without representing pathogenic infection.
Findings in viral CNS infection on peripheral blood count are leukocytosis or leukopenia, atypical lymphocytes (EBV), or eosinophilia (eosinophilic encephalitis) [32]. Serum amylase may be elevated in mumps; cold agglutinins occur in mycoplasma, upon which serologic investigations are indicated for mycoplasma and Chlamydia; infiltrates on chest X-ray in atypical pneumonia should be followed by investigation for Mycoplasma, Legionella or Lymphocytic choriomeningitis virus. HIV testing should be performed for uncertain causes of CNS infection [32].
In fungal meningitis, in immunosuppressed patients, CSF may appear normal, although opening pressure may be high. In cryptococcal meningitis in HIV-positive patients a predominantly lymphocytic pleocytosis with low CSF glucose and high protein levels is common [39]. The encapsulated fungus can be identified by India ink examination of CSF, and serologic detection of cryptococcal antigen is sensitive and relatively specific for CNS cryptococcal infection. Repeat LP and CSF analysis to document CSF sterilization is not indicated routinely [92].
A patient who does not respond clinically after 48 hours of appropriate antimicrobial therapy, especially in cases of penicillin- or cephalosporin-resistant pneumococcal meningitis, and adjunctive dexamethasone therapy, should undergo repeat CSF analysis [92,121].
57.6 Neuroimaging Findings in Central Nervous System Infections
In uncomplicated bacterial meningitis, CT scans are usually sufficient for clinical management to assess cerebral edema, hydrocephalus, and base of skull pathology such as fractures, inner ear infection, or mastoiditis. Other important findings include subdural effusions, brain stem encephalitis (rhombencephalitis) with Listeria infection, and cortical infarcts secondary to vasculitis (20% of cases) [122]. CT or MR venography should be used to diagnose cerebral venous sinus thrombosis. MR DWI shows parenchymal complications of meningitis, such as infarcts of septic vasculitis earlier than other sequences. Encephalitis, cerebritis, and TB are DWI-hyperintense; neurocysticercosis lesions are DWI-hypointense. Toxoplasmosis is variable, whereas lymphoma shows no restriction of water diffusion [122]. MR angiography can suggest vasculitis. Ventriculitis is detected best by T2 fluid-attenuated inversion recovery (FLAIR) images showing periventricular hyperintensity or ependymal enhancement and irregular intraventricular debris [122]. In TBM, basal meningeal enhancement, hydrocephalus, tuberculoma, and infarction are all more common than in bacterial meningitis, where subdural collections are more common [123]. Based on a CT study of 94 children with TBM [123] basal meningeal enhancement or tuberculoma was 89% sensitive and 100% specific for diagnosis of TB. Precontrast hyperdensity in the basal cisterns on cranial MRI may be the most specific radiologic sign of TBM in children [124]. In Lyme disease, multifocal nonenhancing patchy lesions on T2 weighted images (T2WI) can be seen [122]. In acute HSE, FLAIR or T2WI show asymmetrical changes of necrotizing encephalitis in the first 48 hours and may be diagnostic. Diffusion abnormalities disappear within 14 days after symptom onset, while T2 hyperintensities persist [122]. Single photon emission CT (SPECT) may indicate hyperperfusion suggesting inflammation and neuronal injury in the temporal and frontal lobes relatively early, although half of scans are normal in the acute stage, and hyperperfusion is a nonspecific finding [71].
Bacterial abscesses are hyperintense on DWI with reduced apparent diffusion coefficient (ADC) in contrast to nonpyogenic lesions, which are generally hypointense or mixed-signal. Proton MR spectroscopy shows lactate, cytosolic amino acids, and absence of choline [122]. Extra-axial bacterial empyemas appear slightly hyperintense relative to CSF and hypointense to white matter on T1WI and hyperintense on T2WI, distinguishing them from sterile effusions and chronic hematomas [122]. Epidural hematomas can be distinguished from subdural empyema by a hypointense rim representing displaced dura in the former. On DWI, subdural empyema is usually hyperintense, whereas epidural hematoma usually has a low or mixed-signal intensity [122].
57.7 Antimicrobial Therapy
57.7.1 Distribution of Drug Into the Central Nervous System
Antimicrobial distribution into the CNS is influenced by physical barriers, and physiochemical properties of the antimicrobial itself. The major physiochemical factors governing antimicrobial penetration into the CNS are listed in Table 57.2. Only the unbound concentration of drug is available to diffuse across the BBB and blood-CSF barrier. Therefore highly protein-bound agents have limited ability to penetrate the CNS [125].
The tight junctions within the BBB and blood-CSF barrier limit diffusion of large molecular weight substances (>500 daltons). Anti-infectives with larger molecular weights such as vancomycin or amphotericin B have limited ability to penetrate an intact barrier [27,125]. More lipophilic agents (defined by a high octanal-to-water partition coefficient) will penetrate the CNS more readily than hydrophilic compounds. Lipophilicity can also affect the distribution of drugs within the CNS. Highly lipophilic agents accumulate in the lipid-rich brain structures, with a resultant lower concentration in the CSF and extracellular fluid. CSF levels may therefore underestimate overall CNS penetration [125]. The degree of ionization at physiologic pH also affects penetration of the BBB. Most drugs are weak acids or weak bases, and therefore polarity can vary depending on the pH. The pH of blood, CSF, and extracellular fluid changes with infection, and this can influence the polarity and, therefore anti-infective penetration of the CNS [125].
The choroid plexus contains specialized active transcellular transport systems involved in the excretion of drugs across the cerebral vessel wall [27]. During active CNS infection, impaired CSF excretion systems may result in higher CSF concentrations of antibiotics. As inflammation diminishes, antibiotic concentrations may subsequently decrease [28]. Disruption of the BBB during inflammation also affects antibiotic penetration. Hydrophilic agents such as vancomycin rely on passive diffusion through opening of tight junctions in the BBB and therefore have increased penetration during meningeal inflammation [126]. Hydrophilic drugs which use active transport systems such as penicillin and ceftriaxone may have lower concentrations in brain tissue after BBB injury [127].
57.7.2 Timing and Appropriateness of Initial Antimicrobial Therapy
Mortality from delaying antibiotic treatment for CT and LP is estimated at 10 to 20 times the risk of complications associated with lumbar puncture. A prospective multicenter observational study of 156 adults hospitalized for pneumococcal meningitis found three factors independently associated with 3-month mortality: simplified acute physiology score II, isolation of a nonsusceptible strain, and an interval of greater than 3 hours between hospital admission and administration of antibiotics [128]. A retrospective cohort study of 286 adults with community-acquired bacterial meningitis assessed causes of unfavorable outcomes [129]. The major finding was that time to starting appropriate antibiotic treatment relative to the onset of first symptoms and particularly to the onset of consciousness disturbance was significantly delayed in the poor outcome group. Practice guidelines for the management of bacterial meningitis recommend that patients with purulent meningitis receive the first dose of antibiotics as soon as possible, and that antibiotic administration not be delayed for an LP [92]. The appropriate selection of initial antibiotics is also crucial. In a retrospective review of 109 subjects with community-acquired meningitis, 22 subjects did not receive appropriate initial antibiotic coverage, of whom 100% died, as compared with 23% of patients receiving appropriate therapy [130].
Physiochemical property | Effect on CNS penetration |
Lipophilicity | Highly lipophilic drugs more readily penetrate the CNS |
Protein binding | Highly protein bound drugs have reduced CNS penetration |
Molecular weight | Substances >500-800 daltons have reduced ability to penetrate the BBB |
Ionization | Polar, ionized compounds are less likely to cross the BBB. Polarity can vary for many drugs with changes in physiologic pH |
Active transport | Specialized active transport cells in the choroid plexus may excrete drugs across the vessel wall |
Table 57.2. Physiochemical properties influencing antimicrobial penetration into the CNS.
57.7.3 Antimicrobial Selection and Dosing
Meningitis
Empiric therapy of meningitis must consider patient age, immune status, and whether the infection is acquired in the community or nosocomial setting. Recommendations for empiric therapy of meningitis are listed in Table 57.3. Organism-specific recommendations are provided in Table 57.4.
Patient-specific factor | Common pathogens | Preferred regimen | Alternative regimens |
18-50 years | S. pneumoniae, N. meningitidis, H. influenzae (non-immunized patients) | Ceftriaxone1 2 g IV q 12 hrs or cefotaxime2 2 g IV q 6 hrs | Meropenem2 2 g IV q 8 hrs or chloramphenicol3,4 50 to 100 mg/kg/day in 4 divided doses or moxifloxacin3 400 mg IV daily |
plus vancomycin2,5 15 mg/kg IV q 8-12 hrs | plus vancomycin2,5 15 mg/kg IV q 8-12 hrs | ||
>50 years | S. pneumoniae, N. meningitides, L. monocytogenes, gram-negative bacilli | Ampicillin2 2 g IV q 4 hrs | Ampicillin2 2 g IV q 4 hrs or trimethoprim/sulfamethoxazole2 5 mg/kg (TMP-SMX) IV q 6 hrs |
plus vancomycin2,5 15 mg/kg IV q 8-12 hrs | plus vancomycin2,5 15 mg/kg IV q 8-12 hrs | ||
plus ceftriaxone1 2 g IV q 12 hrs or cefotaxime2 2 g IV q 6 hrs | plus meropenem2 2 g IV q 8 hrs or moxifloxacin3 400 mg IV daily | ||
Impaired immunity | L. monocytogenes, gram negative bacilli, S. pneumoniae, N. meningitidis | Ampicillin2 2 g IV q 4 hrs | Ampicillin2 2 g IV q 4 hrs or trimethoprim/sulfamethoxazole2 5 mg/kg (TMP-SMX) IV q 6 hrs |
plus vancomycin2,5 15 mg/kg IV q 8-12 hrs | plus vancomycin2,5 15 mg/kg IV q 8-12 hrs | ||
plus ceftazidime2 2 g IV q 8 hrs or cefepime2 2 g IV q 8 hrs | plus meropenem2 2 g IV q 8 hrs or ciprofloxacin2 400 mg IV q 8 hrs | ||
Neurosurgery, head trauma, cerebrospinal shunt | Staphylococci, gram-negative bacilli, S. pneumoniae | Vancomycin2,5 15 mg/kg IV q 8-12 hrs | Vancomycin2,5 15 mg/kg IV q 8-12 hrs |
plus cefepime2 2 g IV q 8 hrs or ceftazidime2 2 g IV q 8 hrs | plus meropenem2 2 g IV q 8 hrs or ciprofloxacin2 400 mg IV q 8 hrs |
Table 57.3. Recommendations for empiric antimicrobial therapy for bacterial meningitis in adults [131].
1 Dose adjustments may be indicated in patients with combined hepatic and renal insufficiency; 2 Dose adjust for renal insufficiency; 3 Caution in patients with hepatic insufficiency, dose adjustment may be required; 4 Monitor for bone marrow suppression, including leukopenia, thrombocytopenia, and aplastic anemia; 5 Monitoring of serum drug levels is recommended to optimize dosing
Organism | Preferred regimen | Alternative regimen | Duration |
S. pneumoniae (PCN MIC <0.1) | Penicillin G1 4 million units IV q 4 hrs or ceftriaxone2 2 g IV q 12 hrs or cefotaxime1 2 g IV q 6 hrs | Meropenem1 2 g IV q 8 hrs or chloramphenicol3,4 50 to 100 mg/kg/day in 4 divided doses or moxifloxacin3 400 mg IV daily | 10-14 days |
S. pneumoniae (PCN MIC >0.1) (CTX MIC <0.5) | Ceftriaxone2 2 g IV q 12 hrs or cefotaxime1 2 g IV q 6 hrs | Meropenem1 2 g IV q 8 hrs or moxifloxacin3 400 mg IV daily or vancomycin1,5 15 mg/kg IV q 8-12 hrs | 10-14 days |
S. pneumoniae (PCN MIC >0.1) (CTX MIC >0.5) | Vancomycin1,5 15 mg/kg IV q 8-12 hrs plus ceftriaxone2 2 g IV q 12 hrs or cefotaxime1 2 g IV q 6 hrs | Vancomycin1,5 15 mg/kg IV q 8-12 hours plus rifampin3 900-1200 mg IV/PO daily in 2-3 divided doses | 10-14 days |
N. meningitidis | Penicillin G1 4 million units IV q 4 hrs | Ampicillin1 2 g IV q 4 hrs or ceftriaxone2 2 g IV q 12 hrs or cefotaxime1 2 g IV q 6 hrs or chloramphenicol3,4 50 to 100 mg/kg/day in 4 divided doses | 7-10 days |
H. influenzae | Ceftriaxone2 2 g IV q 12 hrs or cefotaxime1 2 g IV q 6 hrs | Chloramphenicol3,4 50 to 100 mg/kg/day in 4 divided doses | 7-10 days |
L. monocytogenes | Ampicillin1 2 g IV q 4 hrs plus gentamicin1,5,6 3-5 mg/kg/day IV in 3 divided doses | Trimethoprim/sulfamethoxazole1 5 mg/kg (TMP) IV q 6 hrs | 21 days |
Group B Streptococcus | Penicillin G1 4 million units IV q 4 hrs or ampicillin1 2 g IV q 4 hrs | Vancomycin1,5 15 mg/kg IV q 8-12 hrs or ceftriaxone2 2 g IV q 12 hrs or cefotaxime1 2 g IV q 6 hrs | 14-21 days |
Enterobacteriaceae, Klebsiella species | Ceftriaxone2 2 grams IV q 12 hrs or cefotaxime1 2 g IV q 6 hrs | Meropenem1 2 grams IV q 8 hrs | 14-21 days |
+/- gentamicin1,5,6 3-5 mg/kg/day IV in 3 divided doses or ciprofloxacin1 400 mg IV q 8 hrs | +/- gentamicin1,5,6 3-5 mg/kg/day IV in 3 divided doses or ciprofloxacin1 400 mg IV q 8 hrs | ||
P. aeruginosa, Acinetobacter species | Cefepime1 2 g IV q 8 hrs or ceftazidime1 2 g IV q 8 hrs | Meropenem1 2 g IV q 8 hrs | 14-21 days |
+/- gentamicin1,5,6 3-5 mg/kg/day IV in 3 divided doses or ciprofloxacin1 400 mg IV q 8 hrs | +/- gentamicin1,5,6 3-5 mg/kg/day IV in 3 divided doses or ciprofloxacin1 400 mg IV q 8 hrs | ||
S. aureus, S. epidermidis (methicillin-sensitive) | Nafcillin2 2 g IV q 4 hrs or oxacillin2 2 g IV q 4 hrs | Vancomycin1,5 15 mg/kg IV q 8-12 hrs +/- rifampin3 900-1200 mg IV/PO daily in 2-3 divided doses | 14-21 days |
S. aureus, S. epidermidis (methicillin-resistant) | Vancomycin1,5 15 mg/kg IV q 8-12 hrs | Linezolid 600 mg IV/PO q 12 hrs | 14-21 days |
+/- rifampin3 900-1200 mg IV/PO daily in 2-3 divided doses |
Table 57.4. Pathogen-specific recommendations for antimicrobial therapy for bacterial meningitis in adults. Reproduced from [127] with permission.
1 Dose adjust for renal insufficiency; 2 Dose adjustments may be indicated in patients with combined hepatic and renal insufficiency; 3 Caution in patients with hepatic insufficiency, dose adjustment may be required; 4 Monitor for bone marrow suppression, including leukopenia, thrombocytopenia, and aplastic anemia; 5 Monitoring of serum drug levels is recommended to optimize dosing; 6 Once daily dosing of gentamicin has been evaluated in an animal model suggesting superior CNS penetration vs. conventional dosing; CTX = Cefotaxime; MIC = Minimum inhibitory concentration; PCN = Penicillin
Before the availability and widespread use of the H. influenzae type B vaccine, Haemophilus influenzae accounted for nearly 50% of bacterial meningitis cases (most being in children) in the United States [1,132]. Now, H. influenzae causes only a small percentage of cases, with the median age of patients with bacterial meningitis shifting from 15 months to 25 years [133]. In the United States and many countries, most cases of community-acquired bacterial meningitis are caused by Streptococcus pneumoniae (47%), followed by Neisseria meningitidis (25%), group B Streptococcus (13%), Listeria monocytogenes (8%), and H. influenzae (7%) [133]. More recently traditionally nosocomial gram-negative organisms (e.g., Klebsiella species, Serratia species, Pseudomonas species, and Acinetobacter species) are becoming increasingly common [134]. Such trends reflect a shift in the epidemiology of meningitis from a disease of the young and otherwise healthy to a disease of the elderly and immunocompromised. The immunocompromised are especially susceptible to meningitis caused by Listeria monocytogenes and gram-negative bacilli.
Of even greater concern is the emergence of resistance of both gram-negative organisms to 3rd and 4th generation cephalosporins and to carbapenems [135-137] and of gram-positive organisms to methicillin (methicillin-resistant Staphylococcus aureus – MRSA) and vancomycin (vancomycin-resistant Enterococcus – VRE). Multidrug resistant (MDR) gram-negative bacteria, including Acinetobacter spp. and Citrobacter spp. [138,139], and gram-positives such as Staphylococcus epidermidis are becoming more common. Even Streptococcus pneumoniae is developing reduced sensitivity to cephalosporins and even carbapenems [140].
In the setting of neurosurgery, head trauma and CSF leak, and cerebrospinal shunts, the most common organisms are S. aureus and gram-negative bacilli. Effective source control is critical, which often necessitates surgical removal of foreign materials. In patients with CSF shunts who are shunt-dependent, removal of the shunt often necessitates placement of a temporary EVD. For TBM, the Infectious Diseases Society of America and American Thoracic Society recommend a similar regimen as for pulmonary TB [141]. An intensive 2-month phase with four drugs (isoniazid, rifampin, pyrazinamide, and ethambutol) is followed by a continuation phase for 6 to 9 months.
Ventriculitis and Cerebrospinal Fluid Shunt Infections
Ventriculitis and EVD and CSF shunt infections frequently involve gram-positive organisms, such as S. epidermidis and S. aureus (including MRSA). Up to 25% are caused by gram-negative organisms. The enteric gram-negative bacilli are of concern in patients who have a ventriculoperitoneal shunt, due to the risk of contamination of the peritoneal end of the catheter [72,81]. Initial broad-spectrum antibiotic selection should include coverage for resistant gram-positive organisms (such as MRSA) and resistant gram-negative organisms (such as Pseudomonas and Acinetobacter species). Vancomycin combined with a cephalosporin with antipseudomonal activity (e.g., cefepime or ceftazidime) or vancomycin plus meropenem are recommended. Infected hardware should be removed, replaced, or externalized when appropriate.
For ventriculitis, there is scientific rationale for installation of antibiotics directly into the ventricles: meningeal inflammation, which enhances the ability of antimicrobials to penetrate the BBB is less pronounced in ventriculitis [142]; and the ventricles can act as a persistent reservoir of infection and inflammation, causing difficulty with eradication and potential blockade of CSF outflow tracts [143]. Under these conditions, the penetration of systemic β-lactams and glycopeptides into the CSF is poor and results in subtherapeutic concentrations in the CSF and high failure rates [144-146].
Intraventricular administration of antibiotics may be necessary in some circumstances in an effort to overcome these difficulties [147,148].
Brain Abscess
The type of pathogen associated with brain abscess depends on patient-specific risk factors. Immunocompromised patients and patients undergoing recent neurosurgery or head trauma (especially penetrating brain injury) are at higher risk [149,150]. Brain abscesses are often polymicrobial (up to 60% of cases), with anaerobic bacteria in up to 49% of cases [151,152]. Frequently isolated organisms include Streptococcus milleri, Bacteroides species, Enterobacteriaceae, and S. aureus. Immunocompromised individuals are at risk for brain abscess from fungi, Nocardia, and Toxoplasma. Optimal treatment involves a combination of antibiotics and surgical intervention to obtain source control. Surgical approaches include stereotactic aspiration or excision. Stereotactic aspiration may be preferred if the abscess is in eloquent brain or an area unsuitable for excision (e.g. brainstem or diencephalon) [153]. Broad spectrum empiric therapy is indicated, the choice of which depends on risk factors for selected organisms (i.e. history, immune status, primary infection site). In immunocompetent patients, initial coverage with vancomycin, ceftriaxone (or cefotaxime), and metronidazole is recommended [150]. Antibiotics should be tailored to the causative organism when microbiologic data become available. Metronidazole often is continued, even if an anaerobic source is not identified on culture because anaerobes are fastidious and have a low culture yield. Patients with recent neurosurgery or head trauma and a presumed nosocomial source of infection should be treated with a third- or fourth-generation cephalosporin with antipseudomonal activity (ceftazidime or cefepime), along with vancomycin (to cover MRSA or methicillin-resistant S. epidermidis) and metronidazole. In HIV-positive patients empiric therapy to cover Toxoplasma with sulfadiazine and pyrimethamine (along with folinic acid) should be considered. Addition of amphotericin B should be considered in neutropenic and post-transplant patients to cover fungal sources, such as Aspergillus, Cryptococcus, and Mucoracae. If Nocardia is suspected, trimethoprim/sulfamethoxazole should be added to the empiric regimen [150,154]. In most cases of brain abscess, 6 to 8 weeks of treatment is required, depending on the clinical and radiographic response.
Epidural Abscess
Empiric treatment of cranial epidural abscess consists of broad-spectrum antibiotics, usually in combination with surgical drainage to prevent progression to subdural empyema. The most common etiologies include streptococci, staphylococci, and anaerobes. Infections are often polymicrobial [72,73]. Initial treatment with vancomycin, ceftriaxone (or cefotaxime), and metronidazole is appropriate in most cases. Substitution of ceftriaxone with ceftazidime, cefepime, or meropenem is recommended where Pseudomonas aeruginosa or other resistant gram-negative nosocomial pathogens are suspected, such as after recent neurosurgery or trauma. Metronidazole is not necessary in regimens containing meropenem which has adequate anaerobic coverage.
Spinal epidural abscess is usually caused by hematogenous spread, with direct seeding of the epidural space. It may also be a complication of adjacent discitis or vertebral osteomyelitis. Other causes of spinal epidural abscess include surgery of the spine, neuraxial anesthesia/analgesia, and LP [72,73,155]. The most common organism involved is S. aureus, occurring in 60% to 90% of cases [155,156]. Other causative organisms include aerobic and anaerobic streptococcal species, and gram-negative organisms such as E. coli and P. aeruginosa [72,73]. Urgent surgical decompression is usually indicated to prevent neurologic deficits. Empiric antimicrobials should be started immediately. In most instances, vancomycin combined with a cephalosporin with antipseudomonal activity (ceftazidime or cefepime) or meropenem is indicated until culture results are available. In selected cases where there are no risk factors for nosocomial drug-resistant organisms, empiric therapy with nafcillin (or oxacillin), combined with a third-generation cephalosporin (ceftriaxone or cefotaxime) may be appropriate. Antibiotic coverage should be narrowed once culture data are available, and treatment should continue for 3 to 4 weeks. In the case of vertebral osteomyelitis or discitis, treatment for 4 to 6 weeks is indicated [72].
Subdural Empyema
Early surgical evacuation in addition to appropriate antibiotic therapy is usually required for successful management. Empiric antibiotic selection for subdural empyema is the same as that for intracranial epidural abscess, as the bacteriology of these infections is identical [75].
Viral Encephalitis
Treatment of viral encephalitis is largely supportive and includes optimization of fluid balance and electrolytes; symptomatic treatment of fever, headache, and nausea; airway protection; management of ICP; and management of seizures. For HSE encephalitis, early treatment (within 2 days of onset) with acyclovir (10 mg/kg intravenously every 8 hours for 14 to 21 days) is associated with improved mortality (70% versus less than 20%) and morbidity [157]. Some other herpes viruses (VZV and herpes B virus) also respond to acyclovir. Therapy usually is started empirically if encephalitis is suspected until diagnostic confirmation is obtained (usually by CSF PCR) [157-159]. Dose adjustment is required in patients with renal insufficiency. Adequate hydration should be maintained during treatment, because acyclovir can cause a crystal nephropathy that is usually reversible. Some recommend repeat CSF examination at completion of treatment and to continue acyclovir if HSV PCR is still positive [32]. Prolonged high dose oral valacyclovir for 3 months after 3 weeks of treatment is also being studied. Oral valacyclovir does not achieve adequate CSF levels to be considered a primary treatment for HSE.
Corticosteroids and mannitol are usually reserved for patients with cerebral edema and intracranial hypertension. However, a nonrandomized retrospective study of 45 subjects with HSE treated with acyclovir found that combination treatment with corticosteroids and acyclovir was an independent predictor of good outcome at 3 months [160]. An experimental encephalitis study showed reduced MRI abnormalities months after infection in animals treated with corticosteroids [161]. Despite the immunosuppressive effects of steroids, they do not enhance viral replication in experimental HSE [162]. For VZV encephalitis, steroids are sometimes used to treat the vasculitic component [32].
CMV encephalitis is an opportunistic pathogen most commonly seen in transplant recipients and other immunosuppressed patients [163]. Current recommendations for both CMV and HHV-6 encephalitis are a combination of ganciclovir (5 mg/kg intravenously every 12 hours) and foscarnet (90 mg/kg intravenously every 12 hours, or 60 mg/kg intravenously every 8 hours) [163-165]. Ganciclovir and foscarnet are associated with severe adverse effects, including renal insufficiency, bone marrow suppression, encephalopathy, and seizures. Both ganciclovir and foscarnet require careful monitoring and dose adjustment in the setting of renal dysfunction [166].
Treatment for severe adenovirus infections have included cidofovir or ribavirin. Patients with life-threatening enterovirus infections have been treated successfully with pleconaril on a compassionate-use basis, and it is deserving of further study [167,168]. Pleconaril is a novel antiviral that inhibits enteroviral replication by integrating into the capsid of picornaviruses and preventing the virus from attaching to cellular receptors and uncoating to release RNA into the cell. Treatment of WNV and other flaviviruses with interferon alpha for 7 days was studied in a randomized, controlled trial of Japanese encephalitis in Vietnamese children, which did not improve outcomes at 3 months [32].
Fungal Central Nervous System Infection
Fungal infections of the CNS are encountered more commonly as opportunistic infections in the immunocompromised. Fungi may cause meningoencephalitis and brain abscess or granuloma. Causative organisms include Cryptococcus neoformans, Coccidioides immitis, Histoplasma capsulatum, Candida albicans, Sporothrix schenkii, Blastomyces dermatidis, Zygomycetes species, and Aspergillus species, with the latter two more commonly causing brain abscess [169]. Optimal treatment of CNS fungal infections is ill defined. The incidence of invasive fungal infections is rising despite the emergence of newer antifungal agents, and outcomes in general are poor [170]. Most treatment regimens consist of an initial induction phase with an amphotericin-based regimen that lasts 2 to 6 weeks, depending on clinical response. This is followed by a chronic suppressive phase with a triazole antifungal with activity against the pathogen being treated (usually fluconazole or voriconazole) that can last from 6 weeks to lifelong suppression. Optimal treatment also should include management of risk factors for fungal disease, such as hyperglycemia, and minimizing immunosuppressant drugs where appropriate.
Although amphotericin B is considered the first-line agent for most CNS fungal infections, it has a large molecular weight and has poor penetration of the BBB. For this reason flucytosine is often added to amphotericin B in the treatment of cryptococcal, Candida, and Aspergillus CNS infections. The dose of amphotericin B deoxycholate (AmB) is 1 mg/kg intravenously daily, combined with flucytosine 25 mg/kg orally every 6 hours. Higher doses (1.5 mg/kg daily), however, are recommended in the setting of mucormycosis [171]. Flucytosine requires dose adjustment in the setting of renal insufficiency, and serum levels should be monitored. Levels greater than 100 mg/ml are associated with an increased risk of bone marrow depression, which can be irreversible and result in death [172,173]. The goal peak flucytosine level is 70 mg/ml to 80 mg/ml (drawn 4 hours after a dose at steady state), and the goal trough level is 30 mg/ml to 40 mg/ml.
In patients with renal insufficiency, a lipid-based amphotericin product is recommended. Both liposomal amphotericin B (L-amB) (5 mg/kg/d) and amphotericin B lipid complex (ABLC) (5 mg/kg/d) have been evaluated in a small number of patients, and they appear to have efficacy similar to conventional amphotericin B [174-176]. The amphotericin B products differ significantly with respect to particle size, electrical charge, and volume of distribution, all of which may influence CNS penetration and antifungal activity. Many animal studies show poor distribution of amphotericin products into the CSF; however, brain tissue concentrations appear to be adequate, and CSF concentrations do not appear to correlate with clinical efficacy [177]. An animal model of C. albicans CNS infection has demonstrated greater antifungal efficacy with AmB and L-AmB over ABLC and amphotericin B colloidal dispersion, but the clinical relevance of these findings has yet to be demonstrated [178].
Voriconazole is a promising therapy for susceptible CNS fungal infections, in particular invasive aspergillosis. Voriconazole penetrates the CSF well with a median CSF-to-plasma ratio of 0.5 [179]. Schwartz and colleagues [180] conducted a retrospective analysis of voriconazole in 81 subjects who had CNS aspergillosis; 35% had a complete or partial response. Thirty-one percent of subjects survived for a median of 390 days and 38% had neurosurgical intervention for the infection, which was associated with improved survival, making it difficult to clearly discern the impact of voriconazole on outcomes. Considering that reported mortality of CNS aspergillosis approaches 100% [181], this study suggests voriconazole is a reasonable option for CNS aspergillosis, and an alternative for other susceptible CNS fungal infections when an amphotericin-based regimen cannot be tolerated. Initial dosing of voriconazole is 6 mg/kg intravenously every 12 hours for two doses, followed by 4 mg/kg intravenously every 12 hours. Voriconazole is metabolized by the hepatic CYP isoenzymes CYP2C9, CYP2C19, and CYP3A4 and is susceptible to numerous drug interactions, with many combinations being contraindicated.
Posaconazole is an extended spectrum triazole antifungal with in vitro activity against Candida, Aspergillus, Zygomycosis, and Fusarium species. In an open-label clinical trial in patients with refractory invasive fungal infection the subset of patients with CNS disease had mostly cryptococcal meningitis and were HIV-positive. Successful outcomes were observed in 14 of 29 (48%) of patients with cryptococcal disease, and 5 of 10 (50%) patients with other fungal infections [182]. Based on this report, and animal studies suggesting efficacy [183,184], posaconazole may be considered as salvage therapy in cryptococcal meningitis where other therapies have failed. Currently, posaconazole is only available as an oral medication (200 mg every 6 hours for 7 days, followed by 400 mg twice daily). It is highly lipophilic, with a very large volume of distribution (1,774 l), and is highly protein bound (>98%). It is an inhibitor of cytochrome P450 3A4, and is therefore subject to numerous clinically significant drug interactions.
Caspofungin and micafungin are the first of a new class of antifungals (echinocandins) that target the fungal cell wall through inhibition of β-1,3 glucan synthesis. Caspofungin is highly protein-bound and has a large molecular weight and a small volume of distribution, all of which indicate poor CNS penetration. From animal data the brain tissue-to-plasma ratio is approximately 0.1 [185]. Hsue and colleagues [186] reported a case of treatment failure associated with the use of caspofungin for meningeal coccidiomycosis. In this report, CSF concentrations were undetectable, despite adequate serum concentrations. In spite of sporadic reports of treatment successes [187,188], these agents cannot be recommended for CNS fungal disease at this time.
Given the poor CNS penetration of AmB and its significant systemic toxicities, there has been interest on the use of intrathecal AmB for treating invasive CNS fungal infections. It can be administered through a reservoir device or as an intraventricular injection, and is typically given in conjunction with systemic amphotericin and flucytosine. Dose ranges for intrathecal AmB range from 0.01 mg to 1.5 mg, and intervals range from daily to weekly [189,190]. Adverse effects associated with intrathecal AmB include arachnoiditis, paraplegia, paresthesias, nausea, vomiting, headache, and back pain. Hydrocortisone (15 mg to 25 mg) can be added to the intraventricular mixture to reduce toxicity. Prophylactic administration of antipyretics and antiemetics may also help alleviate some adverse effects. Although intraventricular AmB is usually reserved for patients who have not responded to systemic therapy, or have relapsed, limited evidence shows that early therapy with both systemic and intraventricular amphotericin B can lead to significant differences in survival, and CSF sterilization compared with systemic therapy alone [191,192].
57.8 Glucocorticoids for Bacterial Meningitis
Several meta-analyses regarding adjunctive corticosteroid therapy have been published, most of which include children as the majority of the studied population [193-200]. A Cochrane collaboration review including 18 clinical trials and 2750 people concluded that adjunctive steroid treatment is associated with a reduction in mortality and hearing loss in all patients (adults and children) with bacterial meningitis without major adverse events [193], a finding that concurs with the recommendations of the Infectious Diseases Society of America [92]. Subgroup analysis for causative organisms showed mortality benefit in patients with S. pneumoniae, and reduction in hearing loss in children with H. influenzae.Routine steroid therapy appears to be justified in most adults although two more recent large randomized controlled clinical trials (RCTs) from Vietnam and Sub-Saharan Africa that evaluated the effectiveness of dexamethasone for adult bacterial meningitis concluded that adjunctive steroids were not associated with better survival or reduction in neurological deficits [201,202].
Despite relatively high dose, much of mortality benefit may be due to reduction in systemic rather than neurologic complications.
The rationale for use of steroids is based on experimental data showing that the inflammatory response in the subarachnoid space contributes significantly to the morbidity and mortality of bacterial meningitis [35]. De Gans and colleagues [203] demonstrated in a large prospective randomized, controlled trial that dexamethasone given before or with the first dose of antibiotic and then every 6 hours for 4 days improved the outcome in adults with acute bacterial meningitis, particularly pneumococcal meningitis, without serious adverse effects. Unfavorable outcomes were reduced from 25% to 15% and mortality from 15% to 7% in dexamethasone-treated subjects. On the basis of this study, the Infectious Diseases Society of America Practice Guideline recommends use of dexamethasone (0.15 mg/kg every 6 hours for 2 to 4 days) in patients with suspected meningitis [92]. Dexamethasone should be continued only in patients with gram-positive diplococci on Gram’s stain or S. pneumoniae in CSF or blood cultures. It should not be given to patients with prior administration of antibiotics.
A major concern has been the rapidly rising incidence of highly resistant S. pneumoniae
requiring combination therapy with vancomycin. Vancomycin penetration into the CNS is largely dependent upon meningeal inflammation, and some experimental studies show that dexamethasone significantly decreases the achievement of therapeutic vancomycin concentrations in the CSF [204,205], resulting in clinical treatment failure in adults [206]. In contrast, more recent data suggest this can be overcome by giving higher doses of vancomycin via continuous infusion (15-mg/kg loading dose followed by 60-mg/kg per day infusion) [207]. Expert opinion recommends adjuvant dexamethasone even in patients with highly resistant pneumococcal isolates [92,208]. There are no data to support adjunctive steroids in patients who have nosocomial and postneurosurgical meningitis, and meningitis related to CSF shunts.
In patients who have TBM, a randomized, double-blind, placebo-controlled study of 545 subjects conducted in Vietnam demonstrated that adjunctive treatment with dexamethasone reduces mortality (31.8% versus 41.3%), but probably does not prevent severe disability (18.2% versus 13.8%) [209]. The prespecified subgroup analysis of patients with HIV demonstrated a nonsignificant reduction in the risk of death, although none were treated with antiretroviral drugs, and the results may not be generalizable to populations with access to antiretroviral treatment. The results of this trial suggest that for non-HIV patients with TBM, 4 to 8 weeks of dexamethasone should be administered (2 to 4 weeks of tapering intravenous steroids followed by tapering oral therapy).
57.9 Management of Complications of Acute CNS Infection
57.9.1 Elevated Intracranial Pressure
The management of elevated ICP in conditions such as bacterial meningitis or encephalitis should follow the same principles as for other etiologies. Osmotic therapy with mannitol or hypertonic saline has not been adequately studied in CNS infections. Small doses of mannitol (0.25 to 0.5 mg/kg) appear to be equally effective to larger doses. If mannitol is used, the serum osmolar gap should be followed to ensure accumulation is not occurring [210]. Hypertonic saline may be required to treat SIADH associated with bacterial meningitis given that low serum osmolarity can worsen cerebral edema. Fever may not only raise ICP but also worsen secondary brain injury and should always be aggressively managed [211]. It is recommended to use surface cooling or endovascular methods as antipyretics and cooling blankets have limited efficacy. Steroids may reduce inflammation in the subarachnoid space and lessen cerebral edema. Effect of steroids on ICP in this condition has not been studied. When patients arrive in the ICU having already received antibiotics, but not steroids, it is of questionable benefit to introduce steroids at this time because much of inflammation with meningitis occurs in response to destruction of bacteria by antibiotics [212]. Mechanical interventions to reduce ICP may be warranted provided that a mass lesion has been excluded and risk for cerebral herniation from CSF drainage is minimal. Options include repeat large volume lumbar puncture, lumbar drain placement, ventriculostomy and decompressive craniectomy. Clinicians should have a low threshold to re-image the brain to rule out hydrocephalus (occurs in 20% of acute bacterial meningitis).
57.9.2 Cerebrovascular Complications
Although the lower limit of safe cerebral perfusion pressure is unknown in CNS infections, CPP should be maintained >60 to 70 mmHg to avoid cerebral ischemia even in patients with intact autoregulation. Due to the high incidence of arterial or venous complications in acute bacterial meningitis, computed tomographic venography (CTV), magnetic resonance angiography (MRA), or magnetic resonance venography (MRV) should be considered in patients who deteriorate or fail to improve with antibiotic therapy. Careful anticoagulation appears safe in patients with cerebral venous sinus thrombosis in the setting of CNS infection [213].
57.9.3 Severe Sepsis and Septic Shock
In patients with severe sepsis or septic shock, early goal-directed therapy including aggressive fluid resuscitation reduces mortality and is not associated with worsened cerebral edema [214,215]. In experimental bacterial meningitis it was demonstrated that fluid restriction can decrease cerebral blood flow and worsen cerebral ischemia [216]. This suggests that hypovolemia and hypotension should be avoided in patients with CNS infection. Preference for systemic vasopressors in the setting of meningitis has not been studied. Norepinephrine appears to improve CBF more effectively than dopamine at least in head-injured patients [217]. Vasopressin has been studied in experimental traumatic brain injury and appears to be equally as effective as phenylephrine for maintaining cerebral perfusion pressure, improved intracranial pressure, and brain tissue oxygenation compared with phenylephrine [218]. Based on combined data from 4 clinical trials of drotrecogin alfa, which found that the median APACHE II score of enrolled patients with meningitis was 22, it appears unlikely that patients with meningitis and septic shock should be treated (scores >25 derive benefit). Furthermore, 5.7% of patients with bacterial meningitis had intracerebral hemorrhage as a complication of drotrecogin alfa compared with 1% control patients [219]. The role of systemic corticosteroids in CNS infection with septic shock is unclear at this time. In this regard it is noteworthy that in a post-hoc analysis of the European Dexamethasone in Adulthood Bacterial Meningitis Study [203], investigating only patients with pneumococcal meningitis who died within 14 days, mortality benefit from steroid therapy was attributable to a beneficial effect on systemic complications with no significant reduction in mortality due to neurologic causes [220].
57.9.4 Intraventricular and Intrathecal Antibiotics
The clinical situations in which intraventricular antibiotics have been studied include: 1) severe meningitis with associated ventriculitis; 2) intraventricular rupture of a purulent brain abscess; and 3) cerebrospinal fluid shunt infections. For the latter, there is reasonable evidence for the instillation of antimicrobial agents into the ventricles in difficult-to-eradicate shunt infections or in patients who cannot undergo surgical management [92]. Success rates of conservative management of patients with CSF shunt infections caused by coagulase-negative Staphylococci appears to be comparable to conventional management with shunt replacement [221].
Intraventricular instillation of most antimicrobials has been viewed as associated with significant toxicity, although most recent reports suggest that intraventricular/intrathecal administration with agents such as polymyxin B, colistin and vancomycin is not associated with severe or irreversible toxicity [222]. Toxicity appears to be dose-related and early reports may have been associated with inappropriate dosing. Moreover, systemic toxicity of the intravenous route of these same agents may be offset by using the CSF route. Exact therapeutic doses and the role of adjuvant systemic or local CSF therapy are not well understood at this time.
There is a positive publication bias in the literature as almost all reports are case reports or small case series and there are no randomized clinical trials of intraventricular antimicrobial use in adult CNS infection. The success rates of CSF sterilization suggest that therapy with intraventricular antimicrobials is a potentially effective treatment against both gram-negative and -positive MDR meningitis as well as fungal meningitis. In a review by Falagas et al. [223] of intraventricular/intrathecal use of polymyxins in 64 patients (31 case reports/series) with gram-negative meningitis, an overall cure rate of 80% was reported including 91% of CNS infections due to Acinetobacter spp. As mentioned earlier, therapy with both systemic and intraventricular amphotericin B in fungal CNS infection can improve survival, and CSF sterilization compared with systemic therapy alone [191,192]. For tuberculous meningitis the addition of intraventricular rifampin or isoniazid has anecdotal reports of efficacy [224,225], whereas multidrug resistant Mycobacterium tuberculosis appears to respond favorably to intraventricular amikacin and levofloxacin [226,227].
Although no antimicrobial agent is approved by the Food and Drug Administration for intraventricular use, commonly used agents (depending on the causative organism) include vancomycin, gentamicin, tobramycin, and polymyxins. The usual dose of intraventricular vancomycin is 10 mg to 20 mg per day, continued for 48-72 hours after CSF cultures become negative. Potential adverse effects of intraventricular vancomycin include ototoxicity, CSF eosinophilia, seizures, altered mental status, and local tissue irritation [147,228]. The usual daily dose of intraventricular gentamicin and tobramycin is 5 mg to 10 mg, and that of amikacin is 10 mg to 20 mg [229,230]. Toxicities for these aminoglycosides are similar to those of vancomycin.
Redosing is determined by calculation of the inhibitory quotient (trough CSF concentration divided by the minimum inhibitory concentration of the agent for the isolated pathogen). This number should exceed 10 to 20 for consistent CSF sterilization [231]. Polymyxins (polymyxin B and colistimethate) have been used in ventriculitis caused by multi-drug resistant Acinetobacter and Pseudomonas species, with the most common adverse effect of meningeal irritation being dose-dependent and reversible [232]. Dosing has varied substantially (5-20 mg of colistimethate daily), and as for all of the above regimens, the optimal duration is unknown. Preservative-free formulations should always be used for intraventricular administration.
57.10 Prophylactic and Periprocedural Antibiotics for Neurosurgical Procedures and External Ventricular Drains
Nosocomial meningitis accounted for 40% of acute adult bacterial meningitis in a review of 493 episodes, of which 81% occurred within 1 month of neurosurgery or head injury [40]. A retrospective study reported an overall meningitis rate of 1.52% [86] in 6243 subjects undergoing craniotomy, of whom 17% did not receive perioperative antibiotics. Independent risk factors for meningitis were CSF leakage, concomitant incision infection, male sex, and duration of surgery. Perioperative antibiotic prophylaxis reduced incidence of incision infections significantly from 8.8% to 4.6%, but did not prevent meningitis. In patients receiving antibiotics, organisms were mainly noncutaneous and tended to be less susceptible to the prescribed prophylactic antibiotics. Meningitis from noncutaneous organisms were associated with a higher mortality rate compared with cutaneous organisms. The investigators recommended use of narrow-spectrum antibiotics for prevention of surgical site infections without any effect on the risk of meningitis.
Ventriculostomy-related infection (VRI) rates are generally 5% to 10%, with a range of 0% to 22% [81]. Definition of CSF infections related to ventriculostomies is important. The following categories of VRI are potentially useful [85]:
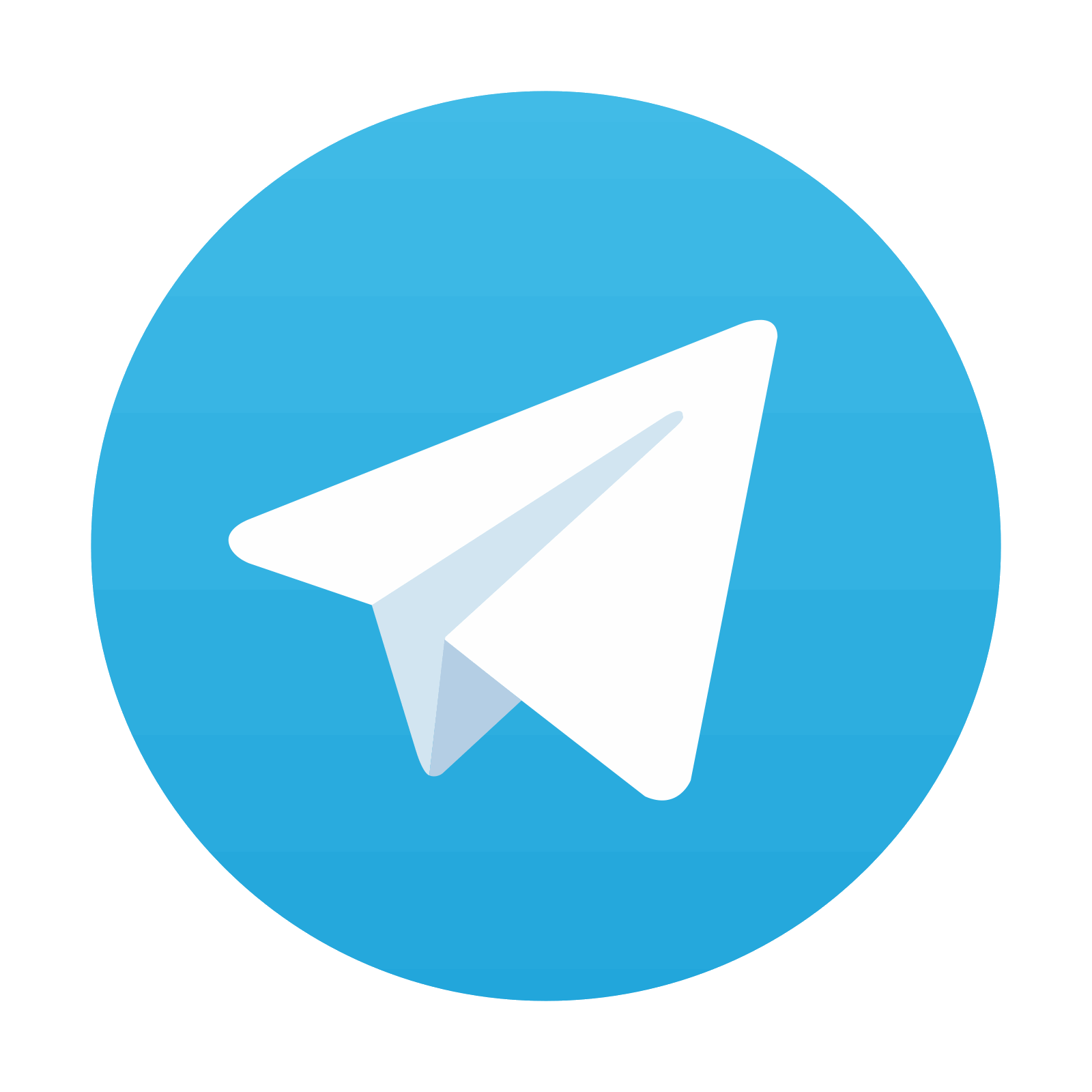
Stay updated, free articles. Join our Telegram channel
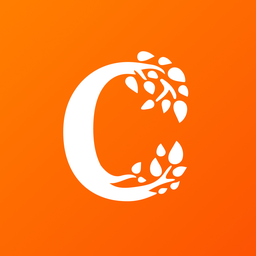
Full access? Get Clinical Tree
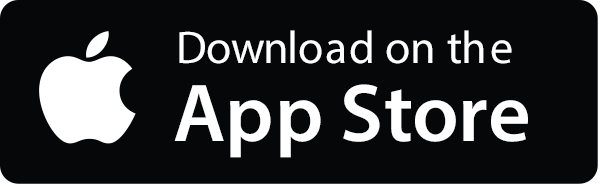
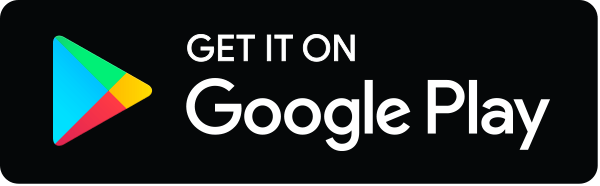