Abstract
While the evidence for many applications of vagus nerve stimulation (VNS) is preliminary, new noninvasive VNS devices have recently been developed and represent significant advancements in VNS therapy. A growing understanding of the mechanism of VNS and a recognition of the association of many medical conditions previously considered distinct offer a new vision of the underlying pathology or pathologies that drive a broad spectrum of symptoms and disease and an innovative way to treat them. VNS addresses the neurotransmitter imbalance that leads to a failure of descending inhibition and abnormal excitation levels, as well as the inflammation that both results from chronic pain by driving the feed-forward loop of neurotransmitter dysfunction and hyperexcitability.
Keywords
Cholinergic antiinflammatory pathway, Central sensitization, Invasive vagus nerve stimulation (iVNS), Metabolic Syndrome, Pain disorders, Vagus nerve stimulation (VNS)
Outline
History and Background of Vagus Nerve Stimulation (VNS) 1551
Neuroanatomy of the Vagus Nerve and Selective Activation by Vagus Nerve Stimulation 1552
Evidence of Neurotransmitter Modulation 1553
The Noradrenergic and Cholinergic Antiinflammatory Pathways, Linking the Autonomic Nervous System With Inflammation 1553
Central Sensitization Through Persistent, Peripheral, Pain Activation, and Inflammation 1556
The Comorbidity of Pain and Somatoform Disorders 1557
Vagus Nerve Stimulation Opposes the Feed-Forward Loop of Inflammation, Neurotransmitter Imbalance, and Neuronal Hyperexcitability 1557
Clinical Evidence of Vagus Nerve Stimulation for the Treatment of Central and Peripheral Pain Disorders and Their Somatoform Comorbidities 1561
Conclusion 1562
References 1562
History and Background of Vagus Nerve Stimulation (VNS)
Stimulation of the carotid sinus and the vagus nerve (VN) by manual manipulation (deep carotid massage) has a long history of use for the treatment of medical conditions ranging from supraventricular tachycardia (SVT) to status epilepticus. The first use of electrical stimulation of the VN was proposed in by Dr. Corning, who used an electrified, external device to stimulate and compress the carotid arteries. Several decades later, vagus nerve stimulation (VNS), decoupled from the vascular compression element (directly applying current to the exposed cervical vagal trunk), was successfully shown to have an effect on chemically induced seizure activity ( ). The mechanism of action (MOA) by which this early attempt at neuromodulation was effective and the development of devices better suited to deliver this bioelectric medical therapy remains an active area of study.
In , Zanchetti et al. pioneered the use of EEG to study the effects of VNS on the cortex in strychnine-poisoned animals. A VNS device for use in humans by Houston-based Cyberonics (now LivaNova) followed in the late 1980s with a US Food and Drug Administration (FDA) approval for refractory epilepsy, being granted in 1997. Subsequent approvals for depression (LivaNova, 2006) and obesity ( ; VBLOC, Enteromedics, 2014) have been granted by the FDA for implanted devices that stimulate the VN. With respect to the latter approval, the stimulation parameters are intended to block activity in the VN, however, for reasons described more fully herein, it is possible that the MOA for the VBLOC’s clinical effects are through activation, not suppression, of vagal activity.
From the late 1990s through today, the availability of approved devices for use in humans has permitted a rapid expansion of case series and formal clinical studies centered on VNS, in indications ranging from depression ( ) to intractable hiccups ( ). Attempts have even been made to apply VNS to the treatment of some of the greatest threats to the modern health systems, metabolic disease ( ) and Alzheimer’s disease ( ).
While the evidence for many applications of VNS is preliminary, a growing understanding of the MOA and a recognition of the association of many medical conditions previously considered distinct, offers a new vision of functional disorders and an innovative way to treat them. These treatments have been facilitated by the introduction of new methods of stimulating the VN noninvasively through activation of the tragus nerve, so-called auricular stimulation (transcutaneous vagal nerve stimulation [tVNS]; Nemos, Cerbomed) or the cervical branches of the VN (noninvasive vagal nerve stimulation [nVNS]; gammaCore, electroCore, LLC).
Neuroanatomy of the Vagus Nerve and Selective Activation by Vagus Nerve Stimulation
The VN contains A-, B-, and C-fibers which differ in diameter and degree of myelination and function. Large, myelinated A-fibers have fast conduction velocities, low electrical thresholds, and carry mostly somatic information. Small, myelinated A-fibers have lower conduction velocities, higher electrical thresholds, and primarily transmit visceral afferent information. Sparsely myelinated B-fibers provide efferent sympathetic and parasympathetic, preganglionic innervation, while small, unmyelinated C-fibers carry afferent visceral information. While there is significant overlap of diameter and conduction velocities for the three fiber types, the electrical thresholds for unmyelinated C-fibers tend to be significantly greater than for myelinated A and B-fibers. The majority of cervical, vagal fibers (60%–80%) are afferent nerve fibers and therefore, carry information from visceral organs to the brain ( ). Clinically relevant, biological effects of VNS, as previously described, have been shown to be due to stimulation of efferent fibers, afferent fibers, or a combination of both.
Early studies on the use of invasive VNS (iVNS) for treatment of epilepsy have implicated afferent A- or B-fibers, but not C-fibers ( ). In a rat seizure study by , destruction of peripheral C-fibers by administration of capsaicin did not affect VNS-induced, seizure suppression. To determine which fibers were activated clinically, compound action potentials (CAPs) were recorded in patients undergoing surgical implantation of the Cyberonics, VNS device ( ). Action potentials (APs) in 21 patients were recorded, and based on conduction velocities, the authors reported that, even with stimulation currents of up to 5 mA (the normal range is 0.25–3.5 mA), only A and B fibers were activated, but not C-fibers. This conclusion is supported by canine studies ( ), in which VNS evoked CAPs elicited firing of A and B fibers at relatively low currents, while C-fiber activation (even at chronaxie [the minimum amount of time needed to stimulate a muscle or nerve fiber, using an electric current twice the strength required to elicit a threshold response]) required 40 to 90 times the activation currents necessary for A fiber activation.
As mentioned earlier, two noninvasive VNS devices have recently been developed and tested in multiple clinical studies. These devices represent significant advancements in VNS therapy as they could potentially provide simple noninvasive treatments that eliminate the expense and morbidity of a surgical procedure. Nemos, from Cerbomed (Germany), stimulates the auricular branch of the VN near the cymba conchae, while gammaCore from electroCore LLC (Basking Ridge, NJ) stimulates the cervical branch of the VN. Several animal and human studies, referenced later in this chapter, have demonstrated that both devices appear to excite the same VN fibers as the Cyberonics (iVNS) device.
In similar functional magnetic resonance imaging (fMRI) studies from the same laboratory, both auricular and cervical VNS produces significant activation of the nucleus tractus solitarius (the first central relay of vagal afferents) and many of its projections (e.g., the spinal trigeminal nucleus, dorsal Raphe nucleus (DRN), locus coeruleus (LC), etc.). Deactivations were observed in the hippocampus and hypothalamus. These responses were not seen in active, sham stimulation of the earlobe in the case of auricular stimulation or in muscle stimulation of the sternocleidomastoid muscle with cervical stimulation ( ) and were similar to changes observed with the iVNS device ( ).
VNS has also been shown, in human studies, to produce a specific pattern of early, EEG, evoked potentials, occurring 3–6 μs after stimulation, regardless of whether the stimulation is auricular, cervical, or iVNS. This suggests activation of the same nerve fibers in all three cases ( ). The fact that these methods of VNS stimulation produce similar evoked potentials doesn’t prove that these are the fibers necessary for producing beneficial clinical effects, but clearly demonstrate noninvasive VNS devices can activate the VN. A computational model of VN activation with cervical stimulation supports the conclusion that iVNS can stimulate A and B, but not C-fibers ( ). In addition, two studies demonstrating equivalence of iVNS and cervical nVNS in the same animal model have been reported ( ).
Evidence of Neurotransmitter Modulation
The development efforts, which ultimately led to the European Union and the United States, FDA approvals of the first implanted VNS device for the treatment of epilepsy, shepherded in the first, broad study period of VNS ( ). The early work by Zanchetti et al. within the space, whose research revealed that VNS modulates cortical activity and alters brain wave activity, has given way to more specific research to identify which nuclei in the brainstem are being activated, what changes in firing rates are seen among various classes of neurons, and what changes in various neurotransmitters occur before and after stimulation is applied.
Shortly after the first FDA approval for VNS, in July of 1997, Schacter and Saper published that the MOA, by which VNS is effective for the treatment of epilepsy, is unknown, but is “clearly different from that of AEDs” (antiepileptic drugs) ( ). As discussed later in this chapter, more recent work looking at the effects of VNS on hyperexcitability phenomena like cortical spreading depression, brings that assertion into question. Nevertheless, the authors have laid out the basic understanding of three significant neural relays from the nucleus tractus solitarius (NTS) (where the afferent VN enters the brainstem) to: (1) the autonomic and somatic centers in the medulla and spinal cord, (2) the reticular activation formation of the medulla, and (3) the ascending projections of the forebrain. It is in this third region that the LC, a small cluster of neurons (typically ∼12,000 neurons in a human ( )) that serves as the primary source of the neurotransmitter (NE), for the CNS, is located. The identification that VNS triggers activation and involvement of the LC, and the fact that the noradrenergic network of fibers emanating from there is one of the most extensive in the CNS, providing noradrenergic transmission throughout the brain, has led to a significant body of work that suggests that the antiepileptic effects of VNS are due to NE. This assertion is supported by observations that VNS’s antiepileptic effects are abolished when the LC is lesioned ( ).
Subsequent observations among implanted epilepsy patients, that VNS can have an effect on patients’ moods (an FDA approval for the treatment of refractory depression with implanted VNS was granted in 2005) led to further study of changes in serotonergic activity. In support of this line of thinking, in the following year, it was reported that the firing rates of serotonin releasing neurons of the DRN were enhanced by VNS, beginning at day 14 of stimulation, and increasing steadily over a 90-day period, while LC firing rates rose nearly immediately, with statistically significant increases observed within the first hour after initiation of stimulation ( ).
An additional mechanism involving the modulation of GABA-ergic transmission has also been proposed. Genetic mutation of the GABA receptor and/or antagonism of GABAergic transmission, are associated with seizure activity. These observations, coupled with clinical successes of drugs that enhance GABAergic activity ( ), have sparked a line of research among the VNS research community. In 2003, Marrosu et al. reported on long-term changes in GABA receptor density among a small group of human subjects after at least 1 year of VNS therapy, when compared with matched, VNS-eligible, nonimplanted controls. Their findings demonstrated that efficacious response to VNS significantly correlates with a normalization of GABA receptor expression, while the controls showed no change in GABA receptor density ( ).
The Noradrenergic and Cholinergic Antiinflammatory Pathways, Linking the Autonomic Nervous System With Inflammation
The role of the sympathetic nervous system (SNS) in the regulation of the immune system has been long appreciated through the activity of the hypothalamic-pituitary-adrenal axis (HPA) and through which corticosteroids (cortisol) and other naturally occurring immunosuppressive compounds are released ( ). In parallel with this understanding, beginning in the 1930s and 1940s, it was observed that a splenectomy could provide relief from severe inflammatory conditions such as rheumatoid arthritis ( ). It was a natural extension of these two lines of thinking, therefore, to attempt to modulate the splenic nerve (an element of the SNS) and identify how the immune system was impacted. The effects of stimulating these neural inputs to the spleen began to be reported as early as the 1960s ( ) ( Fig. 132.1 ).

described the SNS as playing a critical role in a feedback loop that coupled lymphoid organ activity to the CNS. In this model, the efferent arm of the SNS projects to immune system organs, releasing NE from sympathetic nerve terminals in these organs ( ). The role of NE in modulating macrophages and other immune cells in an antiinflammatory direction has been well established ( ). Both endogenous, tonic expression, and volume transmission through extrasynaptic means, i.e., varicosities, have been proposed as a means for maintaining a baseline level of suppression over immune activity ( ).
With respect to the afferent arm of this feedback loop, it has been suggested that peripheral cytokine levels are able to modulate the CNS to alter sympathetic outflow. In fact, two separate groups reported, in 1989 and 1991, that infusion of IL-1 β or IFN- α into the ventricles of the brain causes rapid, significant reductions in peripheral and splenic immune cell activity ( ). To facilitate this activation within the CNS, afferent vagal fibers were proposed as a functional pathway for peripheral cytokine modulation of the CNS ( ).
Further evidence of VN involvement with splenic immune function came when studied the significant peripheral, antiinflammatory effects of semapimod (a compound formerly known as CNI-1493), which, at one point, was believed to inhibit inflammation through inhibition of p38 MAP kinase. Minute quantities of semapimod, were administered intracerebro-ventricular (ICV), just as IL-1β and IFN-α had been used previously. However, unlike the prior thesis of sympathetic pathway involvement, Bernik et al. reversed the assumption of efferent signaling from sympathetic to the parasympathetic (vagus), when it was found that severing of the VN abolished the antiinflammatory effects. Their conclusion was that semapimod was a potent activator of efferent, vagal outflow ( ). had previously demonstrated that electrical stimulation of the distal remains of the severed VN, i.e., the efferent vagal component, was able to trigger antiinflammatory effects, even in the absence of ICV administration of semapimod, IL-1β, or IFN-α. (As will be discussed later, additional studies showed that electrical stimulation of the afferent arms, postvagotomy, were also able to affect the same immune modulation.)
A review of the available literature on this subject strongly suggests that there is broad, albeit not universal, agreement that stimulation of the VN (using appropriate stimulation, signal parameters) generates a splenic nerve-mediated, antiinflammatory effect. Initial proposals to explain the pathway suggest a simple efferent model that is based solely on acetylcholine release (the primary neurotransmitter released by efferent vagal fibers), whereby direct release of acetylcholine and binding to receptors on macrophages suppresses the production of inflammatory cytokines. The specific, efferent pathway was hypothesized to be through a binding of acetylcholine to the α7-nicotinic, acetylcholine receptor (α7nAChR), since the antiinflammatory effect of efferent (postvagotomy) stimulation was lost in α7nAChR knockout animals ( ). This model was quickly narrowed to include only the spleen when it was recognized that irradiation or removal of the spleen abolished the antiinflammatory effects of VNS (but were independently potent antiinflammatory actions). However, the observation that surgical ablation of the splenic nerve and/or depletion of norepinephrine by reserpine eliminated the antiinflammatory effects of VNS required that the hypothesis be amended to something more complicated than simply parasympathetic release of acetylcholine onto splenic macrophages, at least if nerve terminals in the spleen were to be implicated ( ).
To connect efferent VNS to splenic nerve activation, it was postulated that parasympathetic-sympathetic interaction occurs in the celiac ganglion, where vagal fibers and the splenic nerve are in close proximity ( ). In order to incorporate the loss of effectiveness of efferent VNS (post vagotomy) in α7nAChR knockout animals, this interaction was postulated to be mediated (also) through α7nAChRs, with efferent signals in the VN being transmitted to splenic (sympathetic) nerve fibers at the celiac ganglion through α7nAChRs on the splenic nerve.
Subsequent studies in nude, (T cell deficient) β2 knockout animals demonstrated that reconstitution of the T cell population, by infusion of wild type T cells, can restore the efficacy of VNS ( ). This is also suggestive that there is no requirement for an α7nAChR-mediated interaction in the celiac ganglion ( ). This study, which did not isolate the efferent arm of the VN, however, did not take into consideration the possibility of an afferent vagal-efferent sympathetic pathway that is not α7nAChR-mediated. In fact, direct stimulation of the splenic nerve has a strong, antiinflammatory effect in α7nAChR knockout animals ( ), suggesting that if VNS were to maintain its antiinflammatory efficacy in an α7nAChR knockout animal, an afferent vagal-efferent sympathetic pathway, independent of the α7nAChR, might be possible. Subsequent studies have indicated that this is the case ( ). That is, stimulation of the intact VN produces two parallel effects: (1) an efferent effect that is mediated through the celiac ganglion and is mediated by activation of a subset of vagal fibers that release norepinephrine in the vicinity of a T cell population that releases acetylcholine onto the α7nAChRs of the splenic macrophages; and (2) an afferent effect that activates a sympathetic pathway that causes the release of norepinephrine into the spleen in sufficient quantities to directly act on noradrenergic receptors on the macrophages. That is, the efferent arm of VNS appears to be mediated by the α7nAChR, but the afferent arm is not.
Nevertheless, the failure of efferent (postvagotomy) VNS of the distal ends of the vagal bundle to have an antiinflammatory effect in nude animals, which could be restored by infusion of wild type T cells, necessitated further modification of the efferent pathway ( ). More particularly, CD4 + CD25 − Foxp3 − (naïve) T cells, and not CD4 + CD25 + T (regulatory) cells, are required to restore the antiinflammatory effects of VNS. An important difference between naïve and regulatory CD4 + T-cells is the expression of β2-adrenoreceptors (β2ARs). That is, regulatory T-cells lack β2ARs, which may explain their inability to reconstitute the neurosplenic, antiinflammatory mechanism, if they are required to be activated by splenic nerve release of NE. This remains a subject of active study.
Perhaps more importantly, the effect of NE on CD4 + CD25 − naïve T-cells is to promote differentiation into Th1, Th2, and Th17 cells, with the specific pathway being dependent on local cytokine expression. Th2 cells are strongly antiinflammatory, controlling macrophage cytokine production through the production and release of IL-4 and IL-10 ( ). Differentiation into Th2 T-cells is strongly promoted in a low IL-4 environment, which exists in a proinflammatory state, in which macrophages have been activated into an M1 polarization, such as lipopolysaccharide (LPS) binding to the Toll-like receptor (TLR) or other similar proinflammatory event ( ). This is suggestive that the NE release from the splenic nerve, in a proinflammatory environment, causes the differentiation of the CD4 + CD25 − naïve T cells into Th2 cells, strongly releasing IL-4 and IL-10, which suppresses inflammatory activity of local macrophages.
Currently, however, the widely published model that attempts to incorporate this information suggests that splenic nerve release of NE activates locally positioned T cells (CD25 − , or a cell line rapidly differentiated therefrom) to migrate into position near macrophages, to release acetylcholine onto the macrophages to activate their α7nAChRs and down regulate their production of inflammatory cytokines ( ).
This model has yet to be universally accepted, with several research groups already identifying potential failures of the model to account for observations, including the failure of exogenously administered choline to restore the antiinflammatory effect of VNS in nude mice ( ). Continued study is warranted.
A competing model suggests that vagal-efferent fibers release acetylcholine, directly activating α7nAChRs on peripheral (i.e., nonsplenic) T cells which migrate to the spleen and release of acetylcholine onto NE-releasing terminals of the splenic nerve ( ). The antiinflammatory effects in the spleen are thus the result of direct noradrenergic effects on macrophages.
Central Sensitization Through Persistent, Peripheral, Pain Activation, and Inflammation
Central Sensitization is a process by which persistent, peripheral, neural activity (typically associated with pain) generates a lasting imprint on the CNS, whereby future experiences of pain are amplified above what is generally considered appropriate for the triggering event, or whereby the pain no longer requires a peripheral trigger of any kind. In short, prolonged painful stimuli have the potential to alter the CNS in a manner that lowers the threshold of pain perception.
Clifford Woolf explains that pain, in a neuroimmunologic sense, can be broken down into multiple categories ( ). The most basic of these categories is the immediate, nociceptive response designed to prevent or minimize injury when the individual is exposed to or confronted with a threat. Examples of such pain are heat, sharpness, and pressure, and are typically intense, but of short duration. The intensity of the pain experienced in these circumstances is intended to promote withdrawal reflexes and other avoidance responses. These are facilitated by virtue of pathways for this pain that pass through the spinal cord, limiting the need for higher processing. This type of pain is mediated by Aδ-fibers and might be called fast pain .
In this framework, the second category of pain is a consequence of the first, i.e., it is the pain experienced in the short to intermediate interval following an injury. It includes tenderness, achiness, burning, and more prolonged discomfort. This pain may linger for an extended period of time, minutes, hours, or even days, and is often associated with inflammation present during an immune response. This type of pain activates higher emotional centers, is mediated by C-fibers and might be called slow pain . The evolutionary purpose of this hypersensitivity pain is to promote healing and ensure that the individual does not aggravate the damage done by continued activity.
The final category of pain is that which is abnormal and inappropriate. It can be further subdivided into two primary types, that which results from damage to the nervous system (e.g., neuropathic pain) or that which results from improper functioning of the nervous system (dysfunctional pain). Central sensitization is a form of the latter category, but often arises as a consequence of inflammatory pain, and is facilitated by neuroimmune responses.
Persistent pain can be both a consequence of chronic inflammation or it can, itself, give rise to the production of inflammatory mediators such as cytokines and activated immune cells that would not otherwise be present in the inflamed tissue. Although, critical aspects of the healing process prolongation of these activities have the ability to further sensitize nociceptors and, in addition, recruit new nociceptors. Nitric oxide, substance P, prostaglandins, TNF-α, and histamine are examples of chemical mediators of these effects, and in turn, establish a continuous feedback. A full exposition of the complexity of pathologic neuroplasticity, and the specifics of the sensitization process, which includes altered chemical, electrophysiological, and pharmacological states, is beyond the scope of this work ( ). However, from an electrophysiological standpoint, the initiation of the sensitization process involves alterations in nociceptive, primary, order neurons in the dorsal root ganglion (DRG) (first C-fibers, and then abnormally recruited A-fibers) locally (i.e., peripherally), which exhibit lower thresholds for activation and increased firing rates ( ). These afferent, pain fibers first terminate in DRG, where the primary, afferent neurons lie and then terminate in the dorsal horn (DH) of the spinal cord, where they communicate with spinal neurons via synaptic transmission. Excitatory neurotransmitters, such as glutamate and substance P modulate postsynaptic responses with further transmission to regions like the trigeminal ganglion and distal sites via ascending pathways. Normal activation of these ascending pathways is opposed by descending inhibition in the form of elevated thresholds for activation within the higher processing centers (e.g., trigeminal nucleus caudalis and thalamic nuclei). The levels of inhibitory neurotransmitters (e.g., serotonin, acetylcholine, NE, dopamine, and GABA) establish the robustness of this suppressive function, ensuring that only signals strong enough to warrant perception are permitted to pass.
As will be described more fully herein, the combination of bidirectional causation of chronic inflammation and peripherally, sensitized nociception result in abnormally activated inputs to the ascending pathways and prolonged excitation that extends from the DH throughout the ascending pathways. This prolonged excitatory input from nociceptive afferents drives the spinal circuits first and then central pathways to become centrally sensitized, maintaining a chronically excited pain state. Thus, persistent pain leads from and to chronic inflammation, both resulting in a sensitization process within the CNS.
The sensitization process is accompanied by a progressive failure of descending inhibition to restrain, repress, and otherwise filter out ascending pathway excitation. Immune activation associated with persistent pain peripherally, and persistent excitation centrally, leads to proinflammatory cytokine production and modulation of microglial and astrocytic function, contributing to central excitation and the degradation of the levels of inhibitory neurotransmitters that comprise descending inhibition.
The Comorbidity of Pain and Somatoform Disorders
It is an everyday reality of life that injury and sickness can cause pain and discomfort. As a consequence of this readily observable cause and effect relationship, patients and healthcare providers are biased with a deep-rooted assumption that pain arises as the result of an identifiable physical cause, which may be externally driven, such as trauma or infection, or can be internally caused, such as cancer or autoimmune disease. A less frequently encountered reality is, however, that a small, but undeniable fraction of the patient population is afflicted with pain and discomfort that is not so readily explained by a physical cause. In fact, it has been suggested that modern diagnostic means fail in 1 in 10 patients with chronic pain.
With the advent of modern medical record keeping, and the ability to manipulate and study extensive volumes of patient data, the comorbidity of medical conditions that run together with greater than chance frequency has become a focus of interest. An obvious consequence of this interest has been the birth of meta-conditions, such as the one that links obesity, hypertension, insulin resistance (typically Type-2 diabetes), and hyperlipidemia, i.e., Metabolic Syndrome. The observation of the connection among these conditions has led to a search for a common, underlying factor to explain the otherwise mathematically improbable frequency of the co-occurrence of these conditions. That search has led to the recognition of the importance of inflammation as a root cause, likely triggered and/or exacerbated by the activation of TLRs by high concentrations of free fatty acids in hypertrophic, white adipose tissue, and the resulting migration and activation of macrophages into the adipose tissue ( ).
A similar confluence of comorbidity, not yet widely understood to have a common root, exists among chronic pain conditions (e.g., primary headache, temporomandibular disorders (TMDs), and visceral and musculoskeletal pain syndromes), psychiatric disorders (e.g., posttraumatic stress disorder (PTSD), anxiety, and depression), gastric motility disorders (e.g., irritable bowel syndrome and functional dyspepsia), and sleep disorders. Unlike metabolic syndrome, where inflammation was an easily measurable cause, the lack of traditional, peripheral, and physical causation among these conditions has impeded the recognition of a relationship. In retrospect, perhaps it should have been the contrary, with the lack of traditional causation being the common link that is driving them, not to be grouped as separate syndromes, treated by separate specialties (despite their treatment using of many of the same medical therapies), but into a common category with a shared etiology.
This lack of clear and/or sufficient exogenous causation for the pain and discomfort being experienced by individuals strongly suggests the existence of a dysfunctional pain pathway and that an impaired, descending inhibition mechanism is at the root of these patients’ pathologies. Widespread usage (with moderate clinical success) of pharmaceutical agents that modulate neurotransmitter levels further implicates a CNS etiology, including selective serotonin reuptake inhibitors (SSRIs), serotonin norepinephrine reuptake inhibitors (SNRIs), and GABA analogues like gabapentin and pregabalin. Modest to moderately elevated, inflammatory cytokine levels in a high proportion of these individuals, especially in systemic, visceral, and musculoskeletal pain conditions, suggests a chronic inflammatory component to the pathology. The fact that impaired vagal tone and other autonomic dysregulation ( ) present in a high proportion of patients with these symptoms further supports the possibility of VNS being efficacious in treating them.
Vagus Nerve Stimulation Opposes the Feed-Forward Loop of Inflammation, Neurotransmitter Imbalance, and Neuronal Hyperexcitability
As explained earlier, the mechanisms leading to the misperception of head, muscle, joint, gastrointestinal and/or visceral pain (or pain intensity), as well as the disruption of normal sleep patterns and psychiatric conditions, including anxiety and depression disorders, collectively referred to herein as “functional disorders,” often arise as the result of pathologies of the CNS. More particularly, this pathology arises from a failure to properly process peripheral, sensory input. The genesis of this dysfunction may be a prolonged inflammatory reaction, an innate predisposition, allergic or toxic response, or other sensitizing trigger. What is also worthy of note is that these functional disorders are also comorbid with, and/or can serve as risk factors for other pathologies, including stroke, epilepsy, asthma, and worse or worsening outcomes following trauma (i.e., postconcussion syndrome and traumatic brain injury (TBI)) as well as other conditions, not typically considered the result of misprocessing of neural inputs ( ).
To understand this observed connection among seemingly disparate conditions, it is important to bring together the pieces of the puzzle—inflammation, neurotransmitter imbalance, and hyperexcitability—into a comprehensive picture of CNS (and peripheral immune system) dysregulation. These three components form a feed-forward loop, with each element being exacerbated by the other ( Fig. 132.2 ).

Although it is possible to initiate the discussion at any of the three positions, we shall begin with inflammation. CNS immune responses are mediated by a number of different cell types, with microglial cells serving as the principle coordinator of significant, inflammatory responses, as well as reparative functions that help to resolve such events. Like their peripheral counterparts, the macrophages, microglia are mononuclear, phagocytic cells of myeloid derivation. As with macrophages, microglia have traditionally been grouped according to their state of activation, with active microglia of an M1 polarization being cytotoxic, and M2, being the more reparative polarization. Unlike macrophages, however, microglia are nonclonal, and their response to injury is more nuanced, perhaps because of the critical and delicate nature of neuronal tissue in the CNS, when compared with peripheral tissue, that is less so.
In response to a trigger such as injury (and associated production of damage associated molecular patterns, DAMPs) or microbial infection (and the presence of pathogen-associated molecular patterns, PAMPS), microglial cells react rapidly, assuming an M1 polarization state, beginning the production of cytokines. Among the first and most important of these cytokines produced is tumor necrosis factor-alpha (TNF-α), the archetypal, proinflammatory mediator. The expression of this cytokine and others like interleukin-1β (IL-1β) and IL-6 has broad, pleiotropic effects, not only with respect to the activation of other microglia ( ), but in the behavior of other support cells such as astrocytes ( ) and the synthesis and reuptake mechanisms of key neurotransmitters ( ).
As an important example of the latter mechanisms, i.e., the direct disruption in neurotransmitter balance, TNF-α (and other proinflammatory mediators) has a disruptive effect on the pathways for generating serotonin. Specifically, down-regulation of the tryptophan–dependent synthesis of serotonin has been proposed as a consequence of elevated indoleamine,-2,3-dioxygenase (IDO), which is known to alter both serotonergic (and glutamatergic) neuronal activity ( ). Conversely, it has long been known that serotonin, and the serotonin derivative, N -( p -coumaroyl) serotonin, have antiinflammatory effects through the suppression of TNF-α expression in mononuclear myeloid cells ( ).
While disruption of synthesis of serotonin is a potential mechanism by which TNF-α and other inflammatory cytokines alter the impact of this key inhibitory neurotransmitter, perhaps more importantly, these cytokines disrupt transport mechanisms that are necessary for proper, serotonergic homeostasis. More particularly, in parallel with the activation of proinflammatory pathways, these cytokines activate the serotonin transporter SERT, leading to a reduction in extracellular expression of serotonin from key serotonin producing cells of the Raphe nucleus ( ). This enhancement in SERT expression in astrocytes is also modulated by TNF-α ( ).
In addition to the disruption in serotonin homeostasis, TNF-α can substantially interfere with the balance of glutamate through a disruption of proper astrocytic function. Astrocytes are the most numerous, glial-cell variety, with critical functions that include participating in the formation of the BBB and providing support for proper neuronal function. With respect to the latter, astrocytes are tasked with the uptake of glutamate from the synapse, conversion of the glutamate to glutamine, and the return of the glutamine to the presynaptic neuron. TNF-α disrupts astrocytic function through a dual mechanism of directly blocking the uptake of glutamate by astrocytes and altering the release of glutamate by astrocytes ( ). That is, release of TNF-α reduces astroglial, glutamate uptake capacity, and release of IL-1β leads to an inhibition of Ca + waves within the astrocytic syncytium, together fomenting neuronal, glutamate excitotoxicity ( ). In fact, the inflammatory cascade can induce astrocytes to reverse the uptake of glutamate, and actually release glutamate ( ). The central role of TNF-α in this reversal of function was demonstrated most effectively in TNF-α deficient animals, which exhibit a deficient capacity for glutamate release ( ).
VNS’s ability to suppress peripheral, cytokine expression through a deactivation of splenic macrophages, as explained previously, has also been studied with respect to CNS inflammation, the polarization of microglia, and the expression of both cytokines and neurotransmitters. More specifically, with respect to microglial activation and cytokine expression, Ay has reported the effects of implanted, auricular, and cervical, noninvasive VNS in an ischemic stroke model. All three modalities demonstrated an ability to shift microglia from the M1 to M2 polarization, and to significantly suppress the expression of TNF-α ( ).
Referring back to the feed forward loop underpinning critical CNS pathologies, inflammation has the combined effect of reducing the expression of inhibitory neurotransmitters, such as serotonin, and the enhancement of extracellular, excitatory, glutamate levels. This shift in the balance of inhibition and excitation leads to the dual, pronged consequence of impaired descending inhibition (too little inhibitory neurotransmitter) and hyperexcitability (immunoexcitotoxicity). Both of these prongs alter pain pathway function, and render the CNS prone to phenomena that perpetuate and foment the pathology, including cortical spreading depressions (CSDs), disruptions in the BBB, and further activation of immune responses.
CSDs, which have long been asserted as the mechanism underlying migraine aura, and have been shown to be a common occurrence in humans following TBI and ischemic stroke, are a slowly expanding (1–3 mm/s) wave of depolarization that leads to suppressed neuronal function, altered glial function, and a relative loss of maintenance of ion (e.g., Ca ++ and K + ) balance ( ). Disruption of the BBB following a CSD has been asserted in the literature ( ) to act through the upregulation of matrix metalloprotenases (including MMP-9 but not MMP-2), however, opposing conclusions have been drawn by others ( ). There are, however, a series of undeniably profound changes in neural and vascular function that follow in the aftermath of CSDs.
One of the most important of these effects is the activation of microglial cells, and associated elevation in TNF-α expression ( ). Curiously, however, another study looking at the effects of exogenously administered TNF-α on chemically triggered CSDs found that the cytokine had the effect of reducing the amplitude of CSDs in a manner that was dependent on TNFR2, and independent of TNFR1, which indicates that the role TNF-α plays in the resolution of inflammation, which is reliant on TNFR2 binding, may be a the pathway by which this effect was observed ( ). It should be noted here that the broader interpretation that TNF-α suppresses CSDs is not widely accepted, and contradicts other studies that will be discussed herein.
Among the litany of triggers that can initiate CSDs are electrical disturbances (e.g., the injection of electrical charge), chemical triggers (e.g., the delivery of highly concentrated, ionic solutions), and direct physical trauma ( ). The intensity of exposure to such triggers that will initiate a CSD can be thought of as the strength of the barrier or level of resistance the brain has in experiencing the phenomenon. A brain that is considered highly excitable is more susceptible to CSDs. In the case of electrical charge injection, the resistance to CSD initiation can be quantified by establishing the electrical threshold below which the brain is resistant to experiencing a CSD. Measurements of electrical thresholds have also been used to study the effects of AEDs, many of which are believed to owe their therapeutic effects to their ability of enhancing electrical thresholds. It is noteworthy that AEDs typically require several weeks to months of administration before the full benefits of the therapies are clinically expressed.
In addition to the chemicals that are known to initiate CSDs including potassium chloride (KCl) and calcitonin gene related peptide (CGRP), and pharmaceutical agents known to raise electrical thresholds (the aforementioned AEDs, including topiramate), there are naturally occurring compounds that alter the resistance or susceptibility of the brain to CSDs. An important one appears to be serotonin, with recent reports demonstrating that the lesioning of the DRN (a primary locus of serotonergic neurons) results in enhanced CSDs ( ). Further, depletion of serotonin by administration of para -chlorophenylalanine, a tryptophan hydroxylase inhibitor, has been shown to enhance the risk and frequency of CSDs ( ). Not surprisingly, given the previously discussed relationship between TNF-α and serotonin, TNF-α is among the compounds that reduce the barrier to CSD initiation (presumably through the TNFR1 pathway). This is consistent with the observation that TNF-α knockout animals have extraordinarily high electrical thresholds and are nearly impervious to CSD triggers ( ).
A series of studies have been conducted, looking at the effect of VNS (and specifically noninvasive, cervical VNS) on CSDs and electrical thresholds in the brain ( ). The results of the studies have demonstrated that VNS has the ability to raise electrical thresholds in the brain and reduce the frequency and propagation rate of CSDs. VNS also has the ability to reduce levels of TNF-α expressed in brains in which the chemically triggered CSDs were studied. Research into the effects of serotonin and other neurotransmitters are ongoing.
With respect to the expression of neurotransmitters, as previously described, VNS has been shown to elevate activity in several key areas of the brainstem associated with inhibitory neurotransmitters, including the LC (NE), DRN (serotonin), and the periaqueductal gray (GABA). Work by Mark Baker and et al. at the University of Newcastle ( ) and by Jeffrey Lewine at the Mind Research Network in New Mexico ( ) have confirmed fMRI studies that were conducted at Rutgers-UMDNJ. By using either the auricular VNS or the noninvasive cervical VNS device, stimulation also activates the nucleus basalis of Meynert (acetylcholine). Similarly, imaging work has demonstrated the activation of the substantia nigra (dopamine) and the hypothalamus ( ).
With respect to the expression of excitatory neurotransmitters, Oshinsky reported on the effects of noninvasive cervical VNS on glutamate levels in an animal model ( ). The animals, sensitized by repeated central administration of a proinflammatory soup and subsequently triggered by glyceryl trinitrate (GTN), expressed glutamate levels in key regions of the brainstem, 8–10-fold higher than animals not sensitized with inflammatory soup administration. VNS applied for 2 min to the sensitized animals, contemporaneous with the administration of the GTN trigger, prevents excessive expression of glutamate, reducing its levels to that expressed in the naïve animals. In fact, VNS administered even 90 min after the GTN trigger, and after glutamate levels had already risen by 4–5-fold over the levels expressed in the naïve animals, was able to reduce glutamate expression to naïve levels within 20 min. In this model, VNS was able to both prevent (when delivered immediately prior to the GTN administration) and rapidly reduce (when delivered after the elevated expression levels were observed) the expression of glutamate to levels equivalent to the unsensitized control animals.
In summary, the evidence appears to support the thesis that inflammation drives neurotransmitter imbalance and consequent neuronal hyperexcitability. The phenomena of CSD and other sequellae of hyperexcitability lead to enhanced activation of microglia, potential disruption of the BBB, which, in a feed forward manner perpetuates and exacerbates pathology.
VNS appears to have an impact on the inflammation state, tending to suppress microglial activation and reduce cytokine expression. VNS also activates reuptake mechanisms on astrocytes to take up excessive glutamate, and activates nuclei in the brainstem associated with inhibitory, neurotransmitter expression. The suppressive effects on the CNS inflammation state and modulatory impacts on various neurotransmitters’ expression levels are likely the general mechanistic rationale(s) explaining the effect of VNS on electrical thresholds and susceptibility to CSDs. VNS also has a suppressive effect on other consequences of hyperexcitation, including, but not limited to, descending inhibition impairment, habituation deficit, central sensitization, and improper pain processing.
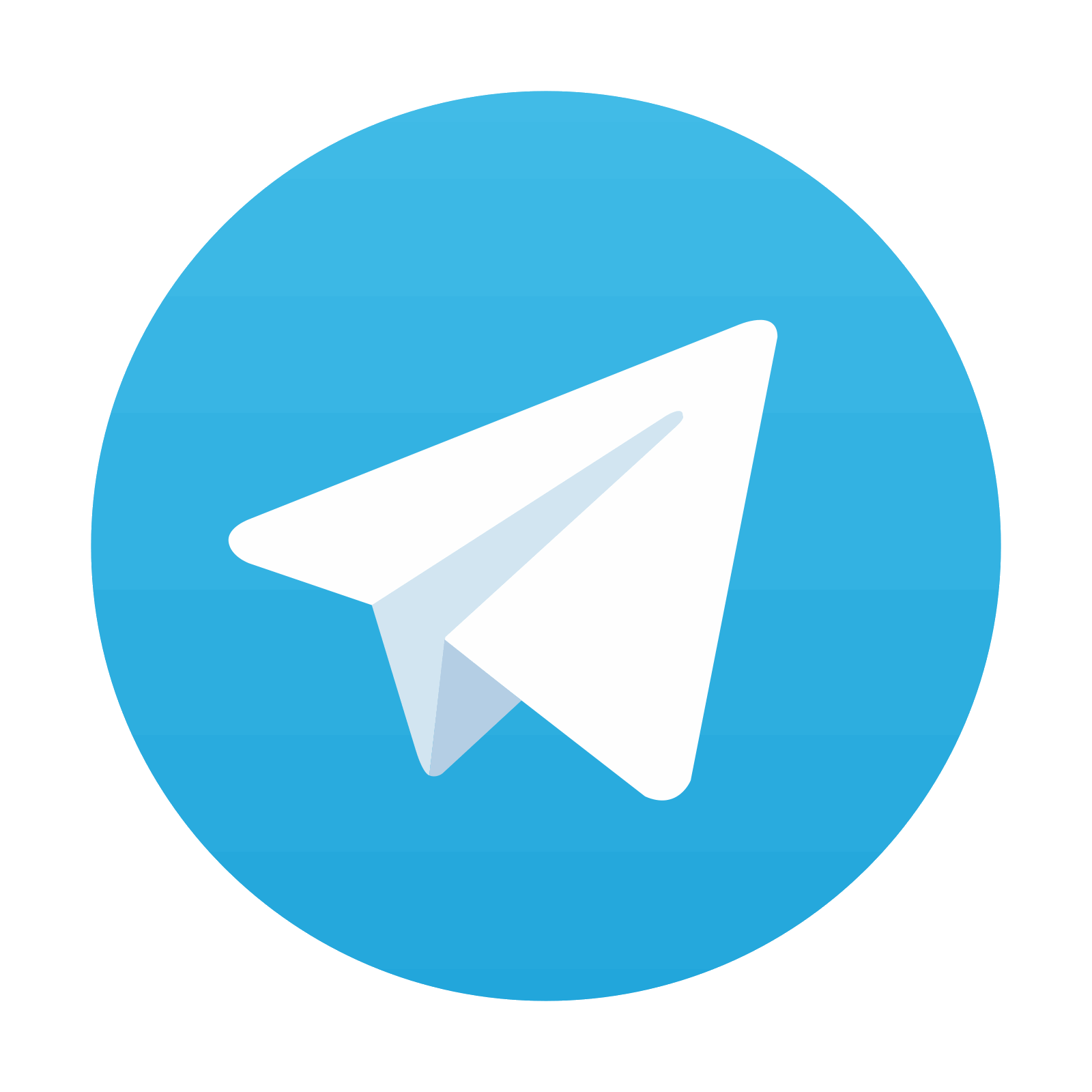
Stay updated, free articles. Join our Telegram channel
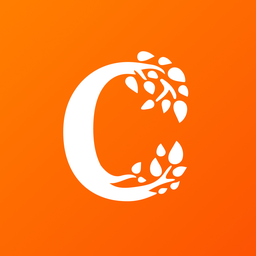
Full access? Get Clinical Tree
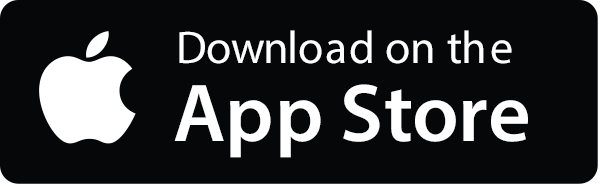
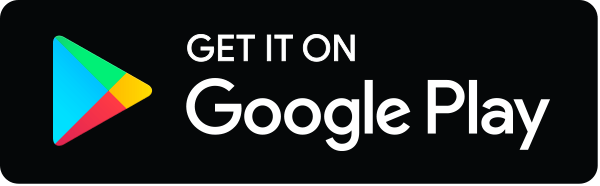